Electrochemical CDR
State of Technology
Overview
Electrochemical carbon dioxide removal technologies from seawater, sometimes called “direct ocean capture” to draw comparisons with direct air capture, encompass both Electrodialytic and Electrolytic techniques that capture and remove dissolved inorganic carbon from seawater (either as CO2 gas or as calcium carbonate), and/or produce a CO2-reactive chemical base, e.g. sodium hydroxide (NaOH), that can be distributed in the surface ocean to ultimately consume atmospheric CO2 and convert it to long-lived, dissolved, alkaline bicarbonate.
In electrodialytic approaches electricity provides the energy to rearrange the most common components of seawater, H2O and NaCl, into acidic (hydrochloric acid; HCl) and basic (sodium hydroxide; NaOH) solutions.1 The acidic solution can then be used to strip dissolved inorganic carbon from seawater in the form of gaseous CO2, or the basic solution can be used to strip the dissolved inorganic carbon by precipitating solid calcium carbonate.2 In addition, the basic component can be selectively added to seawater to draw additional CO2 into the ocean to be stably stored as bicarbonate ions. Electrolytic approaches split water and salt into hydrogen and oxygen and/or chlorine gases, and produce alkaline metal hydroxide (e.g., sodium hydroxide) and acid as byproducts.3 When added to surface seawater, the hydroxide reacts with CO2 in seawater to form dissolved alkaline bicarbonate. The resulting CO2 undersaturation in surface seawater then forces atmospheric CO2 to enter the ocean and be stored largely as long-lived seawater bicarbonate.- House, Kurt Zenz, Christopher H. House, Daniel P. Schrag, and Michael J. Aziz. “Electrochemical Acceleration of Chemical Weathering as an Energetically Feasible Approach to Mitigating Anthropogenic Climate Change.” Environmental Science & Technology 41, no. 24 (December 2007): 8464–70. https://doi.org/10.1021/es0701816.
- Production of calcium carbonate via electrodialysis results in the basic component becoming acidified. This acidic stream may need to be neutralized through addition of a base before being recombined.
- Rau, G.H., Willauer, H.D. & Ren, Z.J. The global potential for converting renewable electricity to negative-CO2-emissions hydrogen. Nature Clim Change 8, 621–625 (2018). https://doi.org/10.1038/s41558-018-0203-0
Technology Readiness
Electrodialytic approaches are already commercial technologies used in applications such as whey demineralization, organic acids recovery, and desalination, among others.1
The electrolytic production of H2, O2/Cl2, hydroxide, and acid is a very mature technology that globally produces some 75 Mt of NaOH per year that is an essential reagent in a variety of important industrial processes (e.g., in food processing, soap production, pulp and paper, pharmaceuticals, etc.).2
An electrolytic approach to precipitate calcium carbonate has recently been proposed3. This approach electrochemically generates alkalinity in order to precipitate calcium carbonate, generating a potentially useful end product from the captured carbon. But, as described above, precipitation of calcium carbonate from seawater acidifies the seawater, reducing the ability of seawater to absorb CO2 from air. This proposed pathway is at a technology readiness level4 of ~2, and thus needs more development before further consideration.
- Bazinet, Laurent; Geoffroy, Thibaud R. 2020. "Electrodialytic Processes: Market Overview, Membrane Phenomena, Recent Developments and Sustainable Strategies" Membranes 10, no. 9: 221. https://doi.org/10.3390/membranes10090221
- Lakshmanan, S. and Murugesan, T. (2014) ‘The chlor-alkali process: Work in Progress’, Clean Technologies and Environmental Policy, 16(2), pp. 225–234.
- Erika Callagon La Plante, Dante A. Simonetti, Jingbo Wang, Abdulaziz Al-Turki, Xin Chen, David Jassby, and Gaurav N. Sant ACS Sustainable Chemistry & Engineering 2021 9 (3), 1073-1089 DOI: 10.1021/acssuschemeng.0c08561
- Mai, T. (2015) Technology Readiness Level, NASA. Brian Dunbar. Available at: http://www.nasa.gov/directorates/heo/scan/engineering/technology/txt_accordion1.html
CDR Potential
- Scalability - Given the immense stocks of dissolved inorganic carbon in seawater (~38,000 Gt1), the theoretical scale of carbon capture from electrochemical methods is limitless for all practical intents and purposes. However, engineering, economic, political, and social considerations are likely to significantly reduce this upper bound. These include, but are not limited to:
-
- Access to low or zero-carbon emissions energy and infrastructure (e.g., desalination plants, offshore wind) with the ability to pump large quantities of seawater into large-scale electrochemical reaction cells, perform electrodialysis or electrolysis, and capture outputs and byproducts. The exact energy and infrastructure needs are pathway-dependent. For instance, electrolysis requires more energy than does electrodialysis, but the relative value of beneficial products (e.g., H2, O2 in electrolysis, HCl in electrodialysis) produced by each process must also be considered2.
- In the cases where electrodialysis is used to produce HCl and strip CO2 gas out of seawater or used to produce NaOH that precipitates calcium carbonate, the cost is high (>$350/ton CO2) because of the cost of pumping seawater3, and in the case of CO2 stripping, the additional cost of safely storing or utilizing the captured CO24
- Costs can be lowered to ~$100/ton CO2 if captured CO2 is stored in the ocean as bicarbonate due to the elimination of seawater pumping costs5
- Electrolytic pathways may offer CDR for $150-100/ton CO2, depending upon whether revenues generated from co-products are used to offset gross costs of CO2 capture6.
- The collective market size for suite of co-products and services offered, including concentrated CO2, H2, O2, HCl, control over critical seawater chemistry parameters like pH and total alkalinity, and CDR.
- Access to low or zero-carbon emissions energy and infrastructure (e.g., desalination plants, offshore wind) with the ability to pump large quantities of seawater into large-scale electrochemical reaction cells, perform electrodialysis or electrolysis, and capture outputs and byproducts. The exact energy and infrastructure needs are pathway-dependent. For instance, electrolysis requires more energy than does electrodialysis, but the relative value of beneficial products (e.g., H2, O2 in electrolysis, HCl in electrodialysis) produced by each process must also be considered2.
Ranges from recent synthesis reports vary with the 2021 NASEM report 7 suggesting a range of 0.1-1.0 Gt CO2/yr with medium confidence, while the 2020 synthesis report from the Energy Futures Initiative suggests a potential range of 1-5 Gt CO2/yr using the "Seawater carbon extraction pathway". 8
- Sequestration Permanence - Electrochemical methods that produce reactive alkaline minerals (e.g., NaOH) will result in additional CO2 from the atmosphere being sequestered in the ocean as bicarbonate ions, which cannot exchange with the atmosphere. This is the same process that results in CO2 sequestration for rock-based forms of ocean alkalinity enhancement. The residence time of bicarbonate ions in the ocean is ~10,000 years, suggesting that electrochemical alkalinity production will generate CO2 sequestration with permanence of ~10,000 years.
Electrochemical methods that produce carbonate mineral products (e.g., ref9) with sequestered carbon are stable on timescales of hundreds-to-thousands of years.
Electrochemical methods which capture and remove CO2 gas from seawater have variable permanence depending upon the storage location for the captured CO2. If the captured CO2 is sequestered in geologic storage, permanence can be long (thousands-to-millions of years)
- Ciais, P., C. Sabine, G. Bala, L. Bopp, V. Brovkin, J. Canadell, A. Chhabra, R. DeFries, J. Galloway, M. Heimann, C. Jones, C. Le Quéré, R.B. Myneni, S. Piao and P. Thornton, 2013: Carbon and Other Biogeochemical Cycles. In: Climate Change 2013: The Physical Science Basis. Contribution of Working Group I to the Fifth Assessment Report of the Intergovernmental Panel on Climate Change [Stocker, T.F., D. Qin, G.-K. Plattner, M. Tignor, S.K. Allen, J. Boschung, A. Nauels, Y. Xia, V. Bex and P.M. Midgley (eds.)]. Cambridge University Press, Cambridge, United Kingdom and New York, NY, USA.
- Campione, A., L. Gurreri, M. Ciofalo, G. Micale, A. Tamburini, and A. Cipollina. “Electrodialysis for Water Desalination: A Critical Assessment of Recent Developments on Process Fundamentals, Models and Applications.” Desalination 434 (May 2018): 121–60. https://doi.org/10.1016/j.desal.2017.12.044.
- Eisaman, Matthew D., Jessy L.B. Rivest, Stephen D. Karnitz, Charles-François de Lannoy, Arun Jose, Richard W. DeVaul, and Kathy Hannun. “Indirect Ocean Capture of Atmospheric CO2: Part II. Understanding the Cost of Negative Emissions.” International Journal of Greenhouse Gas Control 70 (March 2018): 254–61. https://doi.org/10.1016/j.ijggc.2018.02.020.
- Eisaman, Matthew D. “Negative Emissions Technologies: The Tradeoffs of Air-Capture Economics.” Joule 4, no. 3 (March 2020): 516–20. https://doi.org/10.1016/j.joule.2020.02.007
- Rau, G.H. (2021) ‘Testimony to The National Academies of Sciences, Engineering, and Medicine: A Research Strategy for Ocean Carbon Dioxide Removal and Sequestration’ 27-January-2021.
- Erika Callagon La Plante, Dante A. Simonetti, Jingbo Wang, Abdulaziz Al-Turki, Xin Chen, David Jassby, and Gaurav N. Sant ACS Sustainable Chemistry & Engineering 2021 9 (3), 1073-1089 DOI: 10.1021/acssuschemeng.0c08561
- National Academies of Sciences, Engineering, and Medicine. 2022. A Research Strategy for Ocean-based Carbon Dioxide Removal and Sequestration. Washington, DC: The National Academies Press. https://doi.org/10.17226/26278.
- Energy Futures Initiative. “Uncharted Waters: Expanding the Options for Carbon Dioxide Removal in Coastal and Ocean Environments.” December 2020.
- Erika Callagon La Plante, Dante A. Simonetti, Jingbo Wang, Abdulaziz Al-Turki, Xin Chen, David Jassby, and Gaurav N. Sant ACS Sustainable Chemistry & Engineering 2021 9 (3), 1073-1089 DOI: 10.1021/acssuschemeng.0c08561
Environmental Co-benefits
- Electrochemical CDR would likely provide localized reductions in ocean acidification, with expected benefit(s) to marine ecosystems.
- Carbon-negative byproducts from electrochemical CDR can be substituted for more C-intensive sources1:
- Electrolysis: hydrogen gas, chlorine gas, and oxygen gas, as well as hydrochloric acid
- Electrodialysis: hydrochloric acid
- In comparison to rock-based forms of alkalinity enhancement, electrochemical methods may offer:
- Reduced risk of toxicity from metals present in rock-based forms of alkalinity addition2.
- However, the purity of outputs from electrochemical CDR is determined partially by the purity of the input materials to the electrochemical processes, and purer input materials are more expensive.
- Unlike ocean liming and coastal enhanced weathering, electrochemical alkalinity additions will not increase the silica concentration in the ocean and, therefore, may offer a reduced risk of disturbing phytoplankton community dynamics between calcifiers and silicifiers3.
- Rau, Greg H. “Electrochemical Splitting of Calcium Carbonate to Increase Solution Alkalinity: Implications for Mitigation of Carbon Dioxide and Ocean Acidity.” Environmental Science & Technology 42, no. 23 (December 2008): 8935–40. https://doi.org/10.1021/es800366q.
- Hartmann, J., A. J. West, P. Renforth, P. Köhler, C. L. De La Rocha, D. A. Wolf-Gladrow, H. H. Dürr, and J. Scheffran (2013), Enhanced chemical weathering as a geoengineering strategy to reduce atmospheric carbon dioxide, supply nutrients, and mitigate ocean acidification, Rev. Geophys., 51, 113–149, doi:10.1002/rog.20004.
- Bach LT, Gill SJ, Rickaby REM, Gore S and Renforth P (2019) CO2 Removal With Enhanced Weathering and Ocean Alkalinity Enhancement: Potential Risks and Co-benefits for Marine Pelagic Ecosystems. Front. Clim. 1:7. doi: 10.3389/fclim.2019.00007
Environmental Risks
- Risks Shared by Electrolysis and Electrodialysis
- The potential for changes in water column particle concentrations, turbidity, and optical properties if precipitation (inorganic mineral formation) of carbonates occurs due to local increases in alkalinity and pH.
- Mortality of marine life through seawater intake pumps and filters
- Shifts in phytoplankton, invertebrate and vertebrate physiology, competition and/or mortality due to decreases in acidity as CO2 is removed and/or alkalinity is added.1 For example, do the preceding seawater chemistry changes provide an increased competitive advantage for calcifiers over and above the restoration of calcifiers/calcification from ongoing ocean acidification?
- Risks about permanence and impacts of elevated concentrations of bicarbonate in the oceans.
- Risks Specific to Electrolysis
- Chlorine gas production and handling
- Risks Specific to Electrodialysis
- Production of large quantities of acid via electrodialysis that must be safely consumed or neutralized (e.g., via reaction with alkaline minerals).
- For pathways that strip CO2 gas from seawater and sequester the CO2, risks of permanence of storage and potential for leakage
- For pathways that remove dissolved inorganic carbon from seawater as calcium carbonate2, impacts of large quantities of new calcium carbonate on diversity, abundance, and ecosystem function of marine environments
- Renforth, P., and G. Henderson (2017), Assessing ocean alkalinity for carbon sequestration, Rev. Geophys., 55, 636–674, doi:10.1002/2016RG000533.
- Erika Callagon La Plante, Dante A. Simonetti, Jingbo Wang, Abdulaziz Al-Turki, Xin Chen, David Jassby, and Gaurav N. Sant ACS Sustainable Chemistry & Engineering 2021 9 (3), 1073-1089 DOI: 10.1021/acssuschemeng.0c08561
Social Co-benefits
- Localized reductions in ocean acidification, with expected benefit(s) to industries that rely on these localized improvements such as aquaculture and tourism
- Creation of employment in industries producing carbon-negative byproducts from electrochemical CDR 2:
- Electrolysis: hydrogen gas, chlorine gas, and oxygen gas, as well as hydrochloric acid
- Electrodialysis: hydrochloric acid
- OceanNETs Work Package 1: Economic Prospects and Incentives https://www.oceannets.eu/work-package-1-economic-prospects-and-incentives/
- Rau, Greg H. “Electrochemical Splitting of Calcium Carbonate to Increase Solution Alkalinity: Implications for Mitigation of Carbon Dioxide and Ocean Acidity.” Environmental Science & Technology 42, no. 23 (December 2008): 8935–40. https://doi.org/10.1021/es800366q.
Social Risks
- Renforth, P., and G. Henderson (2017), Assessing ocean alkalinity for carbon sequestration, Rev. Geophys., 55, 636–674, doi:10.1002/2016RG000533.
- Erika Callagon La Plante, Dante A. Simonetti, Jingbo Wang, Abdulaziz Al-Turki, Xin Chen, David Jassby, and Gaurav N. Sant ACS Sustainable Chemistry & Engineering 2021 9 (3), 1073-1089 DOI: 10.1021/acssuschemeng.0c08561
Development Gaps and Needs
Addressing Knowledge Gaps
- Electrochemical technologies, as applied to ocean-based CDR, are very new. Because of the lack of proof-of-concept field experiments, it has so far been impossible to characterize benefits, risks, and scaling consideration of OAE in real-world settings (i.e. not benchtop). Controlled field experiments across diverse ecosystems to determine marine chemistry and biology impacts and feedbacks are needed (Develop New Modeling Tools to Support Design and Evaluation, Accelerate Design and Permitting of Controlled Field Trials).
- It is challenging to verify additional CO2 uptake from the atmosphere as a result of electrochemical CDR given the ocean’s dynamic CO2 flux “background state”. New methodologies are needed to observe additional sequestration from the atmosphere into the ocean (Develop New Modeling Tools to Support Design and Evaluation, Develop New In-Water Tools for Autonomous CDR Operations)
- Laboratory experiments are needed across a range of seawater chemistries expected because of expected electrochemical CDR variations in seawater total alkalinity and dissolved inorganic carbon1 to characterize environmental impacts (Accelerate Design and Permitting of Controlled Field Trials).
- Look to the ocean acidification community’s effort to develop standardized protocols for guidance2 as the community builds out standardized protocols and treatments levels for consistency and inter-comparability
- Global, local, and regional predictions of physical, chemical, and biological outcomes and feedbacks of electrochemical CDR from high-resolution models (Develop New Modeling Tools to Support Design and Evaluation)
- Life cycle assessments to calculate net CDR benefits, taking into account all emissions associated with supply chains (Develop CDR Monitoring and Verification Protocols).
- Comments from Dr. Ros Rickaby, ‘Workshop on Ocean-based CDR Opportunities and Challenges, Part 2: Technological and Natural Approaches to Ocean Alkalinity Enhancement and CO2 Removal’ on 27th January 2021
- Gattuso, Jean-Pierre, and Lina Hansson. “European Project on Ocean Acidification (EPOCA): Objectives, Products, and Scientific Highlights.” Oceanography 22, no. 4 (December 1, 2009): 190–201. https://doi.org/10.5670/oceanog.2009.108.
Engineering Challenges and Needs
- Current observational technologies (sensors, ROVs, AUVs, etc.) and modeling tools are not widespread and easily available to support field trials with the necessary spatial and temporal frequency of monitoring and sampling (Develop New In-Water Tools for Autonomous CDR Operations)
- In the case of surface seawater alkalinity addition from electrolysis or electrodialysis, cost-effective and safe methods need to be developed that optimize the distribution, dispersal and dilution of any strong chemical bases to avoid impacts of excessively alkaline (pH>9) waters on marine ecosystems. Dilution may require pumping large amounts of seawater, which need to be optimized for energy and cost (Develop New In-Water Tools for Autonomous CDR Operations).
- Offshore deployment of either electrodialysis or electrolysis would require further development to overcome challenges of working in the ocean (corrosion, biofouling, storms, physical and chemical stress on electrodes, catalysts, and membranes, etc.) (Develop New In-Water Tools for Autonomous CDR Operations).
- Questions remain about how variations in seawater particulate and dissolved organic matter, temperature, and salinity affect electrochemical CDR efficiency (Develop New In-Water Tools for Autonomous CDR Operations).
-
- Methods of handling chlorine gas produced as a byproduct of seawater electrolysis are needed (Develop New In-Water Tools for Autonomous CDR Operations, Improve Understanding of Markets for Co-Products). As an example, chlorine can be used to extract lipids from microalgae 1 and the oil can then be used to produce high density polyethylene 2 hull structures for offshore electrochemical CDR operations. As such, this chlorine management path may lead to a largely self replicating HDPE-based marine CDR infrastructure at the basic materials level. Self replicating offshore infrastructure is highly valuable for DOC operations and the USN is currently 3D printing thick walled HDPE submarines 3 yet offshore electrochemical and biomass CDR reactor hulls can simply be capped off extruded pipes of large diameter and lenght 4, no complex 3D production expense needed.
- Development of feedback control systems that integrate nearby observational data to determine optimal levels of electrochemical CDR (Develop New Modeling Tools to Support Design and Evaluation, Develop New In-Water Tools for Autonomous CDR Operations).
- Identifying and understanding how equipment, energy, and cost scale from benchtop experiments to small field experiments to globally-relevant deployments (Develop New Modeling Tools to Support Design and Evaluation, Develop New In-Water Tools for Autonomous CDR Operations, Develop CDR Monitoring and Verification Protocols, Accelerate RD&D Through New Partnerships)
-
- Garoma, T., Yazdi, R.E. Investigation of the disruption of algal biomass with chlorine. BMC Plant Biol 19, 18 (2019). https://doi.org/10.1186/s12870-018-1614-9
- https://www.mdpi.com/2073-4360/12/8/1641/pdf
- https://3dprint.com/181795/navy-ornl-3d-printed-sub-hull/
- https://www.agru.at/en/applications/agruline/grand-opening-ready-for-the-next-level/
Engineering Challenges and Needs
- Current observational technologies (sensors, ROVs, AUVs, etc.) and modeling tools are not widespread and easily available to support field trials with the necessary spatial and temporal frequency of monitoring and sampling (Develop New In-Water Tools for Autonomous CDR Operations)
- In the case of surface seawater alkalinity addition from electrolysis or electrodialysis, cost-effective and safe methods need to be developed that optimize the distribution, dispersal and dilution of any strong chemical bases to avoid impacts of excessively alkaline (pH>9) waters on marine ecosystems. Dilution may require pumping large amounts of seawater, which need to be optimized for energy and cost (Develop New In-Water Tools for Autonomous CDR Operations).
- Offshore deployment of either electrodialysis or electrolysis would require further development to overcome challenges of working in the ocean (corrosion, biofouling, storms, physical and chemical stress on electrodes, catalysts, and membranes, etc.) (Develop New In-Water Tools for Autonomous CDR Operations).
- Questions remain about how variations in seawater particulate and dissolved organic matter, temperature, and salinity affect electrochemical CDR efficiency (Develop New In-Water Tools for Autonomous CDR Operations).
-
- Methods of handling chlorine gas produced as a byproduct of seawater electrolysis are needed (Develop New In-Water Tools for Autonomous CDR Operations, Improve Understanding of Markets for Co-Products)
- Development of feedback control systems that integrate nearby observational data to determine optimal levels of electrochemical CDR (Develop New Modeling Tools to Support Design and Evaluation, Develop New In-Water Tools for Autonomous CDR Operations).
- Identifying and understanding how equipment, energy, and cost scale from benchtop experiments to small field experiments to globally-relevant deployments (Develop New Modeling Tools to Support Design and Evaluation, Develop New In-Water Tools for Autonomous CDR Operations, Develop CDR Monitoring and Verification Protocols, Accelerate RD&D Through New Partnerships)
-
Public Awareness and Support Are Low
Many of the opportunities and challenges around building public support are not specific to electrochemical CDR, but there are several points of interest specific to electrochemical CDR:
- Electrochemical CDR faces challenges in terms of public perception regarding potential environmental risks not necessarily faced by more “nature-based” approaches, such as coastal blue carbon restoration1 (Accelerate RD&D Through New Partnerships)
- Complexities of electrochemical CDR pathways inhibit understanding and evaluation by non-technical audiences
- There is a great deal of uncertainty and confusion around any ocean-based CDR pathway that relies on adding materials to the ocean. This point applies to both electrochemical alkalinity additions, which return a base to the seawater, and pathways that precipitate out calcium carbonate.
- Earlier ocean iron fertilization experiments2 could offer opportunities to adopt best practices and avoid mistakes made when building public support for electrochemical CDR.
- Electrochemical CDR has the advantage that it can be “turned off” more easily than the other approaches, enabling great control over its application
- Clearer communication strategies need to be developed to respond to the “engineering” narrative of electrochemical CDR.
- Bertram C and Merk C (2020) Public Perceptions of Ocean-Based Carbon Dioxide Removal: The Nature-Engineering Divide? Front. Clim. 2:594194. doi: 10.3389/fclim.2020.594194
- Schiermeier, Q. (2009a). Ocean Fertilization Experiment Draws Fire: Indo-German Research Cruise Sets Sail Despite Criticism. Available online at: https://www. nature.com/news/2009/090109/full/news.2009.13.html
First-Order Priorities
First-Order Priorities
Develop New Modeling Tools to Support Design and Evaluation
High-resolution data-assimilative models that can support real-world testing of electrochemical CDR are required. These modeling tools must:
- Account for complex interactions in the immediate vicinity of the electrochemical CDR and downstream impacts
- Provide four-dimensional (space and time) estimates of biogeochemistry in zone of influence both in the presence and absence of OAE. The difference between these two simulations can be used to inform CDR estimates that account for background variability in the ocean.
- CDR estimates from electrochemical CDR must include estimates of the “opportunity cost” of electrochemical CDR - how did electrochemical CDR shift phytoplankton community composition, production, and export?
To support the design of proof-of-concept field trials, these models should also:
- Provide estimates of the size and scale of biogeochemical modification to the ecosystem from electrochemical CDR, allowing for informed placement of sensors to monitor the field trials
- Be capable of simulating passive tracers (e.g. SF6) to inform whether and how these passive tracers may be useful in field trials (e.g., estimating rates of atmospheric CO2 uptake)
- Inform a prioritized set of predictions to be tested during field trials
Accelerate Design and Permitting of Controlled Field Trials
Field trials for the various electrochemical CDR technologies are urgently needed to test both carbon sequestration potential and environmental impacts (both positive and negative). A series of steps are needed to get to a series of controlled field trials. They include:
Accelerate Design and Permitting of Controlled Field Trials
Field trials for the various electrochemical CDR technologies are urgently needed to test both carbon sequestration potential and environmental impacts (both positive and negative). A series of steps are needed to get to a series of controlled field trials. They include:
Develop New In-Water Tools for Autonomous CDR Operations
A new suite of durable, seagoing technologies are needed to support electrochemical CDR RD&D. Technology development needs include:
- MEPC (1975) Method for calculation of dilution capacity in the ships wake. Submitted jointly by the Netherlands and Norway
- “MAMPEC.” Deltares, 31 Jan. 2017, www.deltares.nl/en/software/mampec/.
- Caserini, S., Pagano, D., Campo, F., Abbà, A., De Marco, S., Righi, D., Renforth, P. and Grosso, M., 2021. Potential of Maritime Transport for Ocean Liming and Atmospheric CO2 Removal. Frontiers in Climate, 3, p.22. https://doi.org/10.3389/fclim.2021.575900
Develop CDR Monitoring and Verification Protocols
Standardized methodologies from third parties to verify uptake of atmospheric CO2 resulting from electrochemical CDR will ultimately need to be developed to enable trading of carbon removal credits. Key first steps to support development of these protocols include:
- Convening experts to review advances from modeling tools (Develop New Modeling Tools to Support Design and Evaluation) and controlled field trials (Accelerate Design and Permitting of Controlled Field Trials) to identify satisfied and outstanding data needs necessary to quantify additional CO2 uptake as a direct result of electrochemical CDR. As advances in electrochemical CDR RD&D are made, the satisfied and outstanding data needs will need to be updated.
- Apply existing12, or develop when necessary, life cycle analysis tools to calculate stored carbon after accounting for emissions from required materials, energy, transportation/dispersal, etc.
- Include aspects of sustained monitoring to verify CDR permanence over long time scales as CDR is scaled.
- Koornneed, J. and Nieuwlaar, E., 2009. Environmental life cycle assessment of CO2 sequestration through enhanced weathering of olivine. Working paper, Group Science, Technology and Society, Utrecht University.
- Hartmann, J., West, A.J., Renforth, P., Köhler, P., De La Rocha, C.L., Wolf‐Gladrow, D.A., Dürr, H.H. and Scheffran, J., 2013. Enhanced chemical weathering as a geoengineering strategy to reduce atmospheric carbon dioxide, supply nutrients, and mitigate ocean acidification. Reviews of Geophysics, 51(2), pp.113-149.
Accelerate RD&D Through New Partnerships
Research, development, and demonstration of electrochemical CDR may be accelerated and strengthened by creating partnerships with key industries/sectors, including:
- Offshore renewable energy production, including wind and others, both as power sources and as integrated CDR platforms
- Coastal industries, including desalination and wastewater treatment facilities, which already have infrastructure for pumping/processing seawater or wastewater for CO2 extraction or alkalinity addition.
- Marine research laboratories that already pump seawater and have expertise, technical equipment and infrastructure to support research and development
- Decommissioned or active offshore oil platforms, wells, and reservoirs as sites for CDR and CO2 sequestration.
- Finfish and shellfish aquaculture where the CDR-produced CO2 and/or alkalinity can be used to optimize chemical conditions, including providing relief from ocean acidification.
Developing and strengthening relationships with partner industries may also help promote public acceptance, as well as potentially offer faster routes to obtaining the necessary permitting.
Improve Understanding of Markets for Co-Products
Electrochemical CDR may generate a host of co-products. Assessing and mapping potential new markets to accommodate these co-products is an important step towards determining the feasibility of electrochemical CDR approaches at scale.
Potential co-products include:
- Hydrogen (H2) gas – as a fuel, energy storage medium, and as a feedstock
- Hydrochloric Acid (HCl) – potentially to refine waste products from silicate mining1
- Oxygen (O2) gas
- Chlorine (Cl2) gas – can be combusted with H2 gas to form HCl
- House, Kurt Zenz, Christopher H. House, Daniel P. Schrag, and Michael J. Aziz. “Electrochemical Acceleration of Chemical Weathering as an Energetically Feasible Approach to Mitigating Anthropogenic Climate Change.” Environmental Science & Technology 41, no. 24 (December 2007): 8464–70. https://doi.org/10.1021/es0701816.
Macroalgae Cultivation and Carbon Sequestration
State of Technology
Overview
- FAO. 2018. The global status of seaweed production, trade and utilization. Globefish Research Programme Volume 124. Rome. 120 pp. License: CC BY-NC-SA 3.0 IGO.
- Thierry Chopin & Albert G. J. Tacon (2020): Importance of Seaweeds and Extractive Species in Global Aquaculture Production, Reviews in Fisheries Science & Aquaculture, DOI: 10.1080/23308249.2020.1810626
- García-Poza, Sara, Adriana Leandro, Carla Cotas, João Cotas, João C. Marques, Leonel Pereira, and Ana M. M. Gonçalves. “The Evolution Road of Seaweed Aquaculture: Cultivation Technologies and the Industry 4.0.” International Journal of Environmental Research and Public Health 17, no. 18 (September 8, 2020): 6528. https://doi.org/10.3390/ijerph17186528.
- Duarte CM, Wu J, Xiao X, Bruhn A and Krause-Jensen D (2017) Can Seaweed Farming Play a Role in Climate Change Mitigation and Adaptation? Front. Mar. Sci. 4:100. doi: 10.3389/fmars.2017.00100
- "Running Tide". https://www.runningtide.com/removing
- GESAMP (2019). “High level review of a wide range of proposed marine geoengineering techniques”. (Boyd, P.W. and Vivian, C.M.G., eds.). (IMO/FAO/UNESCO-IOC/UNIDO/WMO/IAEA/UN/UN Environment/ UNDP/ISA Joint Group of Experts on the Scientific Aspects of Marine Environmental Protection). Rep. Stud. GESAMP No. 98, 144 p.
- Krause-Jensen, D., Duarte, C. Substantial role of macroalgae in marine carbon sequestration. Nature Geosci 9, 737–742 (2016). https://doi.org/10.1038/ngeo2790
- Hughes, Adam D., et al. “Does Seaweed Offer a Solution for Bioenergy with Biological Carbon Capture and Storage?” Greenhouse Gases: Science and Technology, vol. 2, no. 6, 2012, pp. 402–407., doi:10.1002/ghg.1319.
- Moreira, D., Pires, J.C.M., 2016. Atmospheric CO2 capture by algae: Negative carbon dioxide emission path. Bioresource Technology 215, 371–379. https://doi.org/10.1016/j.biortech.2016.03.060
- Zhang, C., Zhang, L., Gao, J., Zhang, S., Liu, Q., Duan, P., Hu, X., 2020. Evolution of the functional groups/structures of biochar and heteroatoms during the pyrolysis of seaweed. Algal Research 48, 101900. https://doi.org/10.1016/j.algal.2020.101900
- Roberts, D., Paul, N., Dworjanyn, S. et al. Biochar from commercially cultivated seaweed for soil amelioration. Sci Rep 5, 9665 (2015). https://doi.org/10.1038/srep09665
- Chia, Wen Yi, et al. “Nature’s Fight against Plastic Pollution: Algae for Plastic Biodegradation and Bioplastics Production.” Environmental Science and Ecotechnology, vol. 4, 2020, p. 100065., doi:10.1016/j.ese.2020.100065.
- Siegel, D. A., DeVries, T., Doney, S. C., & Bell, T. (2021). Assessing the sequestration time scales of some ocean-based carbon dioxide reduction strategies. Environmental Research Letters, 16(10), 104003
- National Academies of Sciences, Engineering, and Medicine 2022. A Research Strategy for Ocean-based Carbon Dioxide Removal and Sequestration. Washington, DC: The National Academies Press. https://doi.org/10.17226/26278
Overview
- FAO. 2018. The global status of seaweed production, trade and utilization. Globefish Research Programme Volume 124. Rome. 120 pp. License: CC BY-NC-SA 3.0 IGO.
- Thierry Chopin & Albert G. J. Tacon (2020): Importance of Seaweeds and Extractive Species in Global Aquaculture Production, Reviews in Fisheries Science & Aquaculture, DOI: 10.1080/23308249.2020.1810626
- García-Poza, Sara, Adriana Leandro, Carla Cotas, João Cotas, João C. Marques, Leonel Pereira, and Ana M. M. Gonçalves. “The Evolution Road of Seaweed Aquaculture: Cultivation Technologies and the Industry 4.0.” International Journal of Environmental Research and Public Health 17, no. 18 (September 8, 2020): 6528. https://doi.org/10.3390/ijerph17186528.
- Duarte CM, Wu J, Xiao X, Bruhn A and Krause-Jensen D (2017) Can Seaweed Farming Play a Role in Climate Change Mitigation and Adaptation? Front. Mar. Sci. 4:100. doi: 10.3389/fmars.2017.00100
- "Running Tide". https://www.runningtide.com/removing
- GESAMP (2019). “High level review of a wide range of proposed marine geoengineering techniques”. (Boyd, P.W. and Vivian, C.M.G., eds.). (IMO/FAO/UNESCO-IOC/UNIDO/WMO/IAEA/UN/UN Environment/ UNDP/ISA Joint Group of Experts on the Scientific Aspects of Marine Environmental Protection). Rep. Stud. GESAMP No. 98, 144 p.
- Krause-Jensen, D., Duarte, C. Substantial role of macroalgae in marine carbon sequestration. Nature Geosci 9, 737–742 (2016). https://doi.org/10.1038/ngeo2790
- Hughes, Adam D., et al. “Does Seaweed Offer a Solution for Bioenergy with Biological Carbon Capture and Storage?” Greenhouse Gases: Science and Technology, vol. 2, no. 6, 2012, pp. 402–407., doi:10.1002/ghg.1319.
- Moreira, D., Pires, J.C.M., 2016. Atmospheric CO2 capture by algae: Negative carbon dioxide emission path. Bioresource Technology 215, 371–379. https://doi.org/10.1016/j.biortech.2016.03.060
- Zhang, C., Zhang, L., Gao, J., Zhang, S., Liu, Q., Duan, P., Hu, X., 2020. Evolution of the functional groups/structures of biochar and heteroatoms during the pyrolysis of seaweed. Algal Research 48, 101900. https://doi.org/10.1016/j.algal.2020.101900
- Roberts, D., Paul, N., Dworjanyn, S. et al. Biochar from commercially cultivated seaweed for soil amelioration. Sci Rep 5, 9665 (2015). https://doi.org/10.1038/srep09665
- Chia, Wen Yi, et al. “Nature’s Fight against Plastic Pollution: Algae for Plastic Biodegradation and Bioplastics Production.” Environmental Science and Ecotechnology, vol. 4, 2020, p. 100065., doi:10.1016/j.ese.2020.100065.
CDR Potential
- Carbon Capture - The ultimate CDR potential of large-scale macroalgal cultivation and sequestration is as yet difficult to determine. Recent consensus reports cite the following as possible carbon dioxide removal potential from macroalgae cultivation and sequestration:
- 0.1 – 1.0 Gt CO2/year (NASEM 2022: A Research Strategy for Ocean-Based Carbon Dioxide Removal and Sequestration)
- 0.1 – 0.6 Gt CO2/ year (NOAA 2022: Carbon Dioxide Removal Research)
Theoretically, macroalgal cultivation and sequestration could be scaled to between one and greater than ten gigatons of CDR per year1, but realized CDR may be much lower due to a number of factors, including:
-
- Competition for space with existing ocean stakeholders – commercial shipping, commercial fishing, indigenous rights, marine protected areas, non-seaweed aquaculture, etc.
- Challenges with expanding to offshore environments – engineering of moorings and farm structures, challenges with operations and maintenance in distant environments2,3- and the likely associated cost increases (at least at first) associated with offshore operations
- Most open ocean environments require nutrient additions to sustain macroalgal yields. If these nutrients come from the deep ocean, they will be accompanied by high CO2 water that can be released and will offset macroalgal carbon sequestration to some degree (which needs to be quantified).4,5
- If macroalgal farms are located closer to the coastal zone, excess anthropogenic nitrogen inputs could support ~3.5 million km2 of macroalgal cultivation, corresponding with an estimated CDR range of 5- 10 Gt CDR annually6, alleviating competition for “natural” nutrient supplies with phytoplankton and alleviating the need for an upwelled supply of nutrients (and the associated risks of releasing CO2 from upwelled deep ocean waters).
- It is hypothesized that macroalgae-based pathways could supply CDR at moderate cost ($25-125 per ton of CO2 removed)7,8,22, but these cost estimates are preliminary and subject to change as macroalgae-based pathways advance in technological readiness9.
- Researchers at UC Irvine are developing a biophysical-economic model to better understand the geophysical, biological, and economic sensitivities of macroalgae-based CDR pathways10
2. Sequestration Permanence -For cultivated macroalgae to contribute to CDR, the carbon captured in the macroalgal tissue needs to be sequestered to prevent its decomposition and return to the atmosphere. Sequestration pathways include:
-
- Intentionally sinking the macroalgae (pre- or post-processing) into the deep ocean (>1000 meters depth) where it can be sequestered from contact with the atmosphere for hundreds to a few thousand years11 (if remineralized in the deep ocean) or for thousands-to-millions of years (if buried in marine sediments).12
- There are natural analogues to this process already. In macroalgal systems, already ~50% of natural macroalgal production breaks off and becomes dissolved or particulate organic matter. Some of the particulate organic matter is transported to the deep ocean, where the carbon is sequestered.13
- Relying on sedimentary burial below or adjacent to the farm (typically more applicable for farms not located over deep water).
- Oceans2050 is working to determine the permanence of burial under coastal farms
- Harvesting the macroalgae for:
- Bioenergy14
- Combining combustion pathways with carbon capture and storage15 (thousands-to-millions of years if stored in a geologic reservoir)
- Pyrolysis, resulting in biochar16,17 (hundreds-to-thousands of years)
- Production of long-lived bioproducts, such as bioplastics, that are capable of permanent (>100 years) sequestration18}
- Bioenergy14
- Intentionally sinking the macroalgae (pre- or post-processing) into the deep ocean (>1000 meters depth) where it can be sequestered from contact with the atmosphere for hundreds to a few thousand years11 (if remineralized in the deep ocean) or for thousands-to-millions of years (if buried in marine sediments).12
3. Differentiating from Avoided Emissions - Macroalgae can provide a number of other services that either reduce or replace greenhouse gas emissions, but do not remove legacy carbon dioxide pollution from the atmosphere. One example of reducing emissions: red algal supplements added to cattle feed may play a key role in reducing enteric methane emissions from cattle by over 50%19,20. Given methane’s potency as a greenhouse gas, such emissions reductions may play an outsized role in slowing planetary temperature increases through the reduction of greenhouse gases. Macroalgae biomass may also be converted into short-lived, high-value bioproducts, such as food and nutritional supplements21. This process may contribute to avoided emissions if it substitutes for carbon-intensive feedstocks, but it does not represent CDR because of the short timescale over which these products sequester carbon before it returns to the atmosphere. Although the focus of this roadmap is on macroalgae pathways for CDR, many of the obstacles faced, development needs, and near-term opportunities for advancement are relevant to these associated pathways for avoided greenhouse gas emissions.
- Energy Futures Initiative. “Uncharted Waters: Expanding the Options for Carbon Dioxide Removal in Coastal and Ocean Environments.” December 2020.
- Bak, Urd Grandorf, Gregersen, Ólavur and Infante, Javier. "Technical challenges for offshore cultivation of kelp species: lessons learned and future directions" Botanica Marina, vol. 63, no. 4, 2020, pp. 341- 353. https://doi-org.oca.ucsc.edu/10.1515/bot-2019-0005
- Lovatelli, A., Aguilar-Manjarrez, J. and Soto, D. (2013). Expanding mariculture farther offshore: technical, environmental, spatial and governance challenges. In: FAO Technical Workshop, 22–25 March 2010, Orbetello, Italy. FAO Fisheries and Aquaculture Proceedings No. 24. FAO, Rome, p. 73.
- Pan, Y., Fan, W., Zhang, D. et al. Research progress in artificial upwelling and its potential environmental effects. Sci. China Earth Sci. 59, 236–248 (2016). https://doi.org/10.1007/s11430-015-5195-2
- Oschlies, A., Pahlow, M., Yool, A., and Matear, R. J. (2010), Climate engineering by artificial ocean upwelling: Channelling the sorcerer's apprentice, Geophys. Res. Lett., 37, L04701, doi:10.1029/2009GL041961.
- Comments from C. Duarte, ‘A Workshop on Ocean-based CDR Opportunities and Challenges Part 3: Ecosystem Recovery & Seaweed Cultivation’. A Research Strategy for Ocean Carbon Dioxide Removal and Sequestration: Workshop Series, Part 3. 2nd February 2021. Accessible at: https://www.nationalacademies.org/event/02-02-2021/a-research-strategy-for-ocean-carbon-dioxide-removal-and-sequestration-workshop-series-part-3
- EFI Report. “Uncharted Waters: Expanding the Options for Carbon Dioxide Removal in Coastal and Ocean Environments.” December 2020.
- Capron ME, Stewart JR, de Ramon N’Yeurt A, Chambers MD, Kim JK, Yarish C, Jones AT, Blaylock RB, James SC, Fuhrman R, Sherman MT, Piper D, Harris G, Hasan MA. Restoring Pre-Industrial CO2 Levels While Achieving Sustainable Development Goals. Energies. 2020; 13(18):4972. https://doi.org/10.3390/en13184972
- TRL definitions: https://www.nasa.gov/directorates/heo/scan/engineering/technology/txt_accordion1.htm
- Sustainable Seaweed Solutions, https://www.ess.uci.edu/~sjdavis/seaweed.html
- “Running Tide”. https://www.runningtide.com/removing
- GESAMP (2019). “High level review of a wide range of proposed marine geoengineering techniques”. (Boyd, P.W. and Vivian, C.M.G., eds.). (IMO/FAO/UNESCO-IOC/UNIDO/WMO/IAEA/UN/UN Environment/ UNDP/ISA Joint Group of Experts on the Scientific Aspects of Marine Environmental Protection). Rep. Stud. GESAMP No. 98, 144 p.
- Krause-Jensen, D., Duarte, C. Substantial role of macroalgae in marine carbon sequestration. Nature Geosci 9, 737–742 (2016). https://doi.org/10.1038/ngeo2790
- Hughes, Adam D., et al. “Does Seaweed Offer a Solution for Bioenergy with Biological Carbon Capture and Storage?” Greenhouse Gases: Science and Technology, vol. 2, no. 6, 2012, pp. 402–407., doi:10.1002/ghg.1319.
- Moreira, D., Pires, J.C.M., 2016. Atmospheric CO2 capture by algae: Negative carbon dioxide emission path. Bioresource Technology 215, 371–379. https://doi.org/10.1016/j.biortech.2016.03.060
- Zhang, C., Zhang, L., Gao, J., Zhang, S., Liu, Q., Duan, P., Hu, X., 2020. Evolution of the functional groups/structures of biochar and heteroatoms during the pyrolysis of seaweed. Algal Research 48, 101900. https://doi.org/10.1016/j.algal.2020.101900
- Roberts, D., Paul, N., Dworjanyn, S. et al. Biochar from commercially cultivated seaweed for soil amelioration. Sci Rep 5, 9665 (2015). https://doi.org/10.1038/srep09665
- Chia, Wen Yi, et al. “Nature’s Fight against Plastic Pollution: Algae for Plastic Biodegradation and Bioplastics Production.” Environmental Science and Ecotechnology, vol. 4, 2020, p. 100065., doi:10.1016/j.ese.2020.100065.
- Roque, B.M., Salwen, J.K., Kinley, R., Kebreab, E., 2019. Inclusion of Asparagopsis armata in lactating dairy cows’ diet reduces enteric methane emission by over 50 percent. Journal of Cleaner Production 234, 132–138. https://doi.org/10.1016/j.jclepro.2019.06.193
- Vijn S. et al.,2020. Key Considerations for the Use of Seaweed to Reduce Enteric Methane Emissions From Cattle. Front. Vet. Sci., 23 December 2020. https://doi.org/10.3389/fvets.2020.597430 https://www.frontiersin.org/articles/10.3389/fvets.2020.597430/full
- Biris-Dorhoi ES, Michiu D, Pop CR, Rotar AM, Tofana M, Pop OL, Socaci SA, Farcas AC. Macroalgae A Sustainable Source of Chemical Compounds with Biological Activities. Nutrients. 2020 Oct 11;12(10):3085. doi: 10.3390/nu12103085. PMID: 33050561; PMCID: PMC7601163.
- National Academies of Sciences, Engineering, and Medicine 2022. A Research Strategy for Ocean-based Carbon Dioxide Removal and Sequestration. Washington, DC: The National Academies Press. https://doi.org/10.17226/26278.
Environmental Co-benefits
- Localized buffering/reductions in ocean acidification due to CO2 uptake. 1,2,3,4,5
- Nutrient remediation and metal uptake in eutrophied, polluted coastal waters 6
- Building macroalgae cultivation facilities near shellfish or fish aquaculture facilities may alleviate negative impacts from such activities9 (e.g., deoxygenation, eutrophication)
- Macroalgae farms may attenuate wave energy 7
- Creation of habitat with resulting nurseries for fish and other marine life 8
- Carbon capture at sea may reduce the demand for terrestrial-based CDR alternatives that compete for land and/or freshwater
- Koweek, D. A., Nickols, K. J., Leary, P. R., Litvin, S. Y., Bell, T. W., Luthin, T., Lummis, S., Mucciarone, D. A., and Dunbar, R. B.: A year in the life of a central California kelp forest: physical and biological insights into biogeochemical variability, Biogeosciences, 14, 31–44, https://doi.org/10.5194/bg-14-31- 2017, 2017.
- Hirsh, H. K., Nickols, K. J., Takeshita, Y., Traiger, S. B., Mucciarone, D. A., Monismith, S., et al. (2020). Drivers of biogeochemical variability in a central California kelp forest: Implications for local amelioration of ocean acidification. Journal of Geophysical Research: Oceans, 125, e2020JC016320. https://doi.org/10.1029/2020JC016320
- Kapsenberg, L, Cyronak, T. Ocean acidification refugia in variable environments. Glob Change Biol. 2019; 25: 3201– 3214. https://doi.org/10.1111/gcb.14730
- Pamela A. Fernández, Pablo P. Leal & Luis A. Henríquez (2019) Co-culture in marine farms: macroalgae can act as chemical refuge for shell-forming molluscs under an ocean acidification scenario, Phycologia, 58:5, 542-551, DOI: 10.1080/00318884.2019.1628576
- Xiao, X., Agustí, S., Yu, Y., Huang, Y., Chen, W., Hu, J., Li, C., Li, K., Wei, F., Lu, Y. and Xu, C., 2021. Seaweed farms provide refugia from ocean acidification. Science of The Total Environment, 776, p.145192, https://doi.org/10.1016/j.scitotenv.2021.145192
- Neveux, N., Bolton, J., Bruhn, A., Roberts, D., Ras, M., 2017. The Bioremediation Potential of Seaweeds: Recycling Nitrogen, Phosphorus, and Other Waste Products. https://doi.org/10.1002/9783527801718.ch7
- Mork, M. (1996). “Wave attenuation due to bottom vegetation,” in Waves and Nonlinear Processes in Hydrodynamics, eds J. Grue, B. Gjevik, and J. E. Weber (Oslo: Kluwer Academic Publishing), 371–382.
- Smale, D.A., Burrows, M.T., Moore, P., O’Connor, N., Hawkins, S.J., 2013. Threats and knowledge gaps for ecosystem services provided by kelp forests: a northeast Atlantic perspective. Ecology and Evolution 3, 4016–4038. https://doi.org/10.1002/ece3.774
- National Academies of Sciences, Engineering, and Medicine 2022. A Research Strategy for Ocean-based Carbon Dioxide Removal and Sequestration. Washington, DC: The National Academies Press. https://doi.org/10.17226/26278.
Environmental Risks
- Macroalgae are known to release bromoform and other halomethanes4,5, and thus, large-scale macroalgae cultivation seems likely to increase the release of these substances. This needs additional research as the natural marine sources of these gases are currently estimated to be responsible for around 9% of stratospheric ozone loss, including depletion due to anthropogenic causes6.
- Production of methane, nitrous oxide, and other potentially hazardous gases by the macroalgae
- The potential for CO2 outgassing from pumping deep water to the surface (artificial upwelling) to supply needed nutrients for the macroalgae3.
- Ecological and biogeochemical (especially acidification and hypoxia) impacts in the deep sea from sinking large quantities of macroalgae into the deep ocean
- Such effects are likely to be location-specific
- Impacts to biodiversity and ecosystem function from large-scale cultivation operations, including1,2:
- Enhanced disease and parasite risk
- Alteration of population genetics
- Introduction of non-native species into new environments
- Enhancement in epiphytic calcifiers that could offset carbon sequestration through calcification-induced CO2 release
- Reduced phytoplankton production in and around large macroalgae farms due to competition for nutrients and light
- Ecological and biogeochemical (especially acidification and hypoxia) impacts in the deep sea from sinking large quantities of macroalgae into the deep ocean
- Such effects are likely to be location-specific
- Changes in light and nutrient availability (including possible changes in ocean albedo)
- In near-coastal zones, changes in circulation patterns due to drag by the seaweed could change residence time in nearshore environments
- Changes in coastal residence time may affect the prevalence and intensity of harmful algal blooms (HABs)
- Entanglement of marine megafauna (e.g., whales)
- Campbell, Iona, et al. “The Environmental Risks Associated With the Development of Seaweed Farming in Europe - Prioritizing Key Knowledge Gaps.” Frontiers in Marine Science, vol. 6, 2019, doi:10.3389/fmars.2019.00107.
- Campbell I, Macleod A, Sahlmann C, Neves L, Funderud J, Øverland M, Hughes AD and Stanley M (2019) The Environmental Risks Associated With the Development of Seaweed Farming in Europe - Prioritizing Key Knowledge Gaps. Front. Mar. Sci. 6:107. doi: 10.3389/fmars.2019.00107
- Pan, Y., Fan, W., Zhang, D. et al. Research progress in artificial upwelling and its potential environmental effects. Sci. China Earth Sci. 59, 236–248 (2016). https://doi.org/10.1007/s11430-015-5195-2
- Carpenter, L. J., et al. “Air-Sea Fluxes of Biogenic Bromine from the Tropical and North Atlantic Ocean.” Atmospheric Chemistry and Physics, vol. 9, no. 5, 2009, pp. 1805–1816., https://doi.org/10.5194/acp-9-1805-2009.
- Mehlmann, Melina, et al. “Natural and Anthropogenic Sources of Bromoform and Dibromomethane in the Oceanographic and Biogeochemical Regime of the Subtropical North East Atlantic.” Environmental Science: Processes & Impacts, vol. 22, no. 3, 2020, pp. 679–707., https://doi.org/10.1039/c9em00599d.
- Tegtmeier, S., Ziska, F., Pisso, I., Quack, B., Velders, G. J. M., Yang, X., and Krüger, K.: Oceanic bromoform emissions weighted by their ozone depletion potential, Atmos. Chem. Phys., 15, 13647–13663, https://doi.org/10.5194/acp-15-13647-2015, 2015.
Social Co-benefits
- Job Creation: Seaweed farming could also present new job opportunities for fishers whose work is threatened by climate change4.
- Value-Added Products: High-value bioproducts (pigments, lipids, proteins, etc) can replace more carbon-intensive alternatives in feed, food, fuel, and other commodities.
- Duarte CM, Wu J, Xiao X, Bruhn A and Krause-Jensen D (2017) Can Seaweed Farming Play a Role in Climate Change Mitigation and Adaptation? Front. Mar. Sci. 4:100. doi: 10.3389/fmars.2017.00100
- Campbell I, Macleod A, Sahlmann C, Neves L, Funderud J, Øverland M, Hughes AD and Stanley M (2019) The Environmental Risks Associated With the Development of Seaweed Farming in Europe - Prioritizing Key Knowledge Gaps. Front. Mar. Sci. 6:107. doi: 10.3389/fmars.2019.00107
- Pan, Y., Fan, W., Zhang, D. et al. Research progress in artificial upwelling and its potential environmental effects. Sci. China Earth Sci. 59, 236–248 (2016). https://doi.org/10.1007/s11430-015-5195-2
- https://atlanticseafarms.com/pages/meet-our-farmers
Social Risks
- Competition for Space: Competition for space with existing ocean stakeholders – commercial shipping, commercial fishing, indigenous rights, marine protected areas, non-seaweed aquaculture, etc.
- Potential for entanglement of macroalgae cultivation equipment with shipping, commercial fishing gear, aquaculture farms etc, particularly if the macroalgae farm is free floating
- Financing: Financing any CDR approach brings with it the risk of creating inequity and decreasing social welfare4. With macroalgae cultivation, this may be especially applicable to coastal dwelling communities who rely on the ocean for their livelihoods, food, and cultural meaning.
- Campbell, Iona, et al. “The Environmental Risks Associated With the Development of Seaweed Farming in Europe - Prioritizing Key Knowledge Gaps.” Frontiers in Marine Science, vol. 6, 2019, doi:10.3389/fmars.2019.00107.
- Campbell I, Macleod A, Sahlmann C, Neves L, Funderud J, Øverland M, Hughes AD and Stanley M (2019) The Environmental Risks Associated With the Development of Seaweed Farming in Europe - Prioritizing Key Knowledge Gaps. Front. Mar. Sci. 6:107. doi: 10.3389/fmars.2019.00107
- Pan, Y., Fan, W., Zhang, D. et al. Research progress in artificial upwelling and its potential environmental effects. Sci. China Earth Sci. 59, 236–248 (2016). https://doi.org/10.1007/s11430-015-5195-2
- Cooley, Sarah R., et al. “Sociotechnical Considerations about Ocean Carbon Dioxide Removal.” Annual Review of Marine Science, vol. 15, no. 1, 2023, pp. 41–66., https://doi.org/10.1146/annurev-marine-032122-113850.
Development Gaps and Needs
Addressing Knowledge Gaps
- Proof-of-concept field experiments have not been conducted in open ocean conditions to test growth rates, sequestration potential, and environmental impacts of macroalgal CDR pathways (Accelerate Design and Permitting of Controlled Field Trials)
- Siting analyses are needed to identify optimal nutrient, light, and wave conditions for growth; as well as potential conflicts with other marine industries (such as the NOAA Coastal Aquaculture Siting and Sustainability toolkit) (Develop New Modeling Tools to Support Design and Evaluation)
- A suite of tools and methodologies to estimate productivity, carbon capture, export, and sequestration, including direct and remote sensing approaches1 needs to be built (Develop New Modeling Tools to Support Design and Evaluation, Measure the Scale and Impacts of CDR via Macroalgae Sinking, Develop New In-Water Tools for Autonomous CDR Operations).
- For sinking pathways, the challenges of following the carbon from source (farm) to deposition (seafloor) in energetically active environments (horizontal and vertical currents, turbulent areas, etc.)
- We do not understand enough about the net CDR benefit from a life cycle perspective (Develop New Modeling Tools to Support Design and Evaluation, Accelerate Design and Permitting of Controlled Field Trials, Develop New In-Water Tools for Autonomous CDR Operations, Develop CDR Monitoring and Verification Protocols)
- Challenges exist verifying additional CO2 uptake from the atmosphere to the ocean in a dynamic background as a result of macroalgae cultivation and sequestration CDR pathways (Develop New Modeling Tools to Support Design and Evaluation, Accelerate Design and Permitting of Controlled Field Trials, Develop New In-Water Tools for Autonomous CDR Operations)
- Inclusion of various macroalgal CDR into integrated assessment models to simulate and predict complex, global responses to macroalgal CDR. Examples include changes in CO2 fluxes in other ecosystems via teleconnections (connections in Earth processes and non-continuous geographic regions, which are often caused by processes not immediately apparent from first principles), and to estimate permanence via the various macroalgal CDR pathways (Develop New Modeling Tools to Support Design and Evaluation)
- Increased knowledge base regarding the physiology (including heat tolerance) and genomics of a broader array of potential cultivars – beyond the ten most common - to understand their growth and carbon sequestration potential is needed (Develop New Modeling Tools to Support Design and Evaluation, Accelerate Design and Permitting of Controlled Field Trials)
- Better understanding of how large-scale macroalgae cultivation affects the partitioning of carbon between particulate and dissolved phases, and its implications for CDR (Develop New Modeling Tools to Support Design and Evaluation, Accelerate Design and Permitting of Controlled Field Trials)
- Bell TW, Nidzieko NJ, Siegel DA, Miller RJ, Cavanaugh KC, Nelson NB, Reed DC, Fedorov D, Moran C, Snyder JN, Cavanaugh KC, Yorke CE and Griffith M (2020) The Utility of Satellites and Autonomous Remote Sensing Platforms for Monitoring Offshore Aquaculture Farms: A Case Study for Canopy Forming Kelps. Front. Mar. Sci. 7:520223. doi: 10.3389/fmars.2020.520223
Engineering Challenges and Needs
- Advances are needed in offshore mooring design, as well as viable, durable, and cost-effective farming systems for offshore cultivation technology1 (Develop New In-Water Tools for Autonomous CDR Operations)
- Current observational technologies (sensors, ROVs, AUVs, etc.) and modeling tools are not widespread or easily available to support field trials with the necessary spatial and temporal frequency of monitoring and sampling (Develop New In-Water Tools for Autonomous CDR Operations)
- Technologies to accelerate macroalgal sinking are not well explored or understood (Accelerate Design and Permitting of Controlled Field Trials, Develop New In-Water Tools for Autonomous CDR Operations)
- Wave-powered devices (both for electrical power and upwelling) are in their infancy (Develop New In-Water Tools for Autonomous CDR Operations)
- Upwelling systems must be designed and tested to access deeper water nutrients and keep them in the euphotic zone where they can support macroalgal growth (as opposed to immediately sinking out of the euphotic zone)
- Harvesting and processing technologies that minimize environmental impact and energy use are needed (Develop New In-Water Tools for Autonomous CDR Operations)
- More efficient means of drying the harvested crop (for non-sinking pathways)
- Technologies to scale shore-based hatcheries to produce more juvenile kelp ready for outplanting are needed (Develop New In-Water Tools for Autonomous CDR Operations)
- Bak, Urd Grandorf, Gregersen, Ólavur and Infante, Javier. "Technical challenges for offshore cultivation of kelp species: lessons learned and future directions" Botanica Marina, vol. 63, no. 4, 2020, pp. 341-353. https://doi.org/10.1515/bot-2019-0005
Building a Global Workforce
- Training, skills development, and technology transfers are needed globally to expand available workforce for macroalgal cultivation and sequestration (Accelerate RD&D Through New Partnerships, Broaden Funding Base for RD&D, Growing & Maintaining Public Support)
- Stable, increased research and development funding to build capacity in this sector, such as has been seen with the ARPA-E MARINER program in the US (Broaden Funding Base for RD&D)
Building a Seaweed Carbon Market
- Certification standards for macroalgal carbon sequestration are needed to support developing markets for macroalgae CO2 sequestration (Develop CDR Monitoring and Verification Protocols)
- Scalable business models and markets to support demand for the multitude of potential products that can be derived from macroalgae (nutritional supplements, high value food items, additives, biochar, bioenergy, etc.) are needed to support this industry (Accelerate RD&D Through New Partnerships, Broaden Funding Base for RD&D, Growing & Maintaining Public Support)
Public Awareness and Support Are Low
Many of the obstacles and needs around building and maintaining public support are not specific to macroalgal cultivation and sequestration, but there are a few points of interest specific to macroalgal cultivation pathways (Growing & Maintaining Public Support):
- Industrial-scale farms could have a negative public connotation (e.g. corn fields/monoculture in the ocean)
- If genetically modified macroalgae were to be used to increase cultivation yields and sequestration potential, especially in the context of industrial-scale macroalgae farms, that might be perceived negatively by the public given public views on genetically modified organisms
- Public perceptions of sinking macroalgal may differ from perceptions about conversion of cultivated macroalgae into high value bio products
First-Order Priorities
Develop New Modeling Tools to Support Design and Evaluation
High-resolution data-assimilative models are needed to support real-world testing of macroalgal CDR pathways. These modeling tools must:
- Account for complex interactions in the vicinity of the farm and downstream impacts12
- Provide four-dimensional (space and time) estimates of biogeochemistry in zone of influence both in the presence and absence of macroalgae cultivation. The difference between these two simulations can be used to inform CDR estimates that account for background variability in the ocean.
- CDR estimates from macroalgae must include estimates of the “opportunity cost” of macroalgal carbon sequestration (how much production and export would have occurred in natural phytoplankton communities in the absence of a macroalgal farm?) as well as any enhancements to marine ecosystem productivity due to the presence of a macroalgal farm (is phytoplankton production enhanced above background levels due to the presence of macroalgae farms?).
To support the design of proof-of-concept field trials, these models should also:
- Provide estimates of the size and scale of biogeochemical modification to the ecosystem from macroalgae cultivation, allowing for informed placement of sensors to monitor the field trials.
- Be capable of simulating passive tracers (e.g. SF6) to inform whether and how these passive tracers may be useful in field trials (e.g., estimating rates of atmospheric CO2 uptake).
- Inform a prioritized set of predictions to be tested during field trials.
- van der Molen, J., Ruardij, P., Mooney, K., Kerrison, P., O’Connor, N.E., Gorman, E., Timmermans, K., Wright, S., Kelly, M., Hughes, A.D., Capuzzo, E., 2018. Modelling potential production of macroalgae farms in UK and Dutch coastal waters. Biogeosciences 15, 1123–1147. https://doi.org/10.5194/bg-15-1123-2018
- Coastal Dynamics Laboratory, https://faculty.sites.uci.edu/davis/methodology/
Accelerate Design and Permitting of Controlled Field Trials
Proof-of-concept field trials are urgently needed to test both cultivation and sequestration technologies, as well as the full array of impacts. Field trials are needed to test predictions regarding:
- Cultivation yields and their dependence on species, ocean basin, nutrient availability, and farm design among others
- Performance of deep-water moorings
- Impacts to pelagic marine ecosystems
- Performance of harvesting technologies
- Additional CO2 uptake from the atmosphere into the macroalgae
- https://oceanvisions.org/our-programs/macroalgaeresearchframework/
Measure the Scale and Impacts of CDR via Macroalgae Sinking
Specific field experiments around the impacts of sinking seaweed in the deep ocean are urgently needed. Given the extensive amount of macroalgae cultivated and harvested in the coastal zone, we have more advanced knowledge of the scale impacts of natural accretion into sediments. We now need a global research effort around the fate and impacts of sinking seaweed in the deep ocean. To advance this agenda we need to convene scientists, engineers, seaweed farmers and more to:
- Identify existing deep-sea observatories, facilities, and vehicles to accelerate research on the fate of macroalgal carbon intentionally sunk in the deep ocean
- Develop a standardized list of biological indicators to measure during field trials to facilitate intercomparison between field trials
- Collaborate with existing farms to conduct field trials. This may be especially important for offshore farms that may be more representative of the open ocean conditions necessary to scale cultivation to achieve globally relevant CDR.
- Evaluate the potential to conduct sinking trials with portions of the supply of floating Sargassum patches to evaluate CDR and environmental impacts of sinking. Globally, there are ~10 gigatons of carbon in these Sargassum patches1, much of which is otherwise destined to end up on beaches, where it may become an environmental nuisance and will decompose, returning its carbon to the atmosphere. This may also serve as a way of generating public support for macroalgae CDR given the considerable social, economic, and environmental issues caused by Sargassum patches.
- Review oceanographic data from past cruises, coastal observing systems, and buoy data to acquire needed physical, chemical, and biological information to assess site potential for field experiments.
- Data from pilot studies investigating the effects of kelp on local mitigation of ocean acidification (e.g., in the state of Washington, USA) may also provide useful information on macroalgae growth rates/carbon sequestration rates, as well as environmental co-benefits and risks.
Measure the Scale and Impacts of CDR via Macroalgae Sinking
Specific field experiments around the impacts of sinking seaweed in the deep ocean are urgently needed. Given the extensive amount of macroalgae cultivated and harvested in the coastal zone, we have more advanced knowledge of the scale impacts of natural accretion into sediments. We now need a global research effort around the fate and impacts of sinking seaweed in the deep ocean. To advance this agenda we need to convene scientists, engineers, seaweed farmers and more to:
- Identify existing deep-sea observatories, facilities, and vehicles to accelerate research on the fate of macroalgal carbon intentionally sunk in the deep ocean
- Develop a standardized list of biological indicators to measure during field trials to facilitate intercomparison between field trials
- Collaborate with existing farms to conduct field trials. This may be especially important for offshore farms that may be more representative of the open ocean conditions necessary to scale cultivation to achieve globally relevant CDR.
- Evaluate the potential to conduct sinking trials with portions of the supply of floating Sargassum patches to evaluate CDR and environmental impacts of sinking. Globally, there are ~10 gigatons of carbon in these Sargassum patches1, much of which is otherwise destined to end up on beaches, where it may become an environmental nuisance and will decompose, returning its carbon to the atmosphere. This may also serve as a way of generating public support for macroalgae CDR given the considerable social, economic, and environmental issues caused by Sargassum patches.
- Review oceanographic data from past cruises, coastal observing systems, and buoy data to acquire needed physical, chemical, and biological information to assess site potential for field experiments.
- Data from pilot studies investigating the effects of kelp on local mitigation of ocean acidification (e.g., in the state of Washington, USA) may also provide useful information on macroalgae growth rates/carbon sequestration rates, as well as environmental co-benefits and risks.
- Gouvêa LP, Assis J, Gurgel CFD, Serrão EA, Silveira TCL, Santos R, Duarte CM, Peres LMC, Carvalho VF, Batista M, Bastos E, Sissini MN, Horta PA. Golden carbon of Sargassum forests revealed as an opportunity for climate change mitigation. Sci Total Environ. 2020 Aug 10;729:138745. doi: 10.1016/j.scitotenv.2020.138745. Epub 2020 Apr 17. Erratum in: Sci Total Environ. 2021 Apr 15;765:144696. PMID: 32498159.
Measure the Scale and Impacts of CDR via Macroalgae Sinking
Specific field experiments around the impacts of sinking seaweed in the deep ocean are urgently needed. Given the extensive amount of macroalgae cultivated and harvested in the coastal zone, we have more advanced knowledge of the scale impacts of natural accretion into sediments. We now need a global research effort around the fate and impacts of sinking seaweed in the deep ocean. To advance this agenda we need to convene scientists, engineers, seaweed farmers, and more to:
- Identify existing deep-sea observatories, facilities, and vehicles to accelerate research on the fate of macroalgal carbon intentionally sunk in the deep ocean
- Develop a standardized list of biological indicators to measure during field trials to facilitate intercomparison between field trials
- Collaborate with existing farms to conduct field trials. This may be especially important for offshore farms that may be more representative of the open ocean conditions necessary to scale cultivation to achieve globally relevant CDR.
- Evaluate the potential to conduct sinking trials with portions of the supply of floating Sargassum patches to evaluate CDR and environmental impacts of sinking. Globally, there are ~10 gigatons of carbon in these Sargassum patches1, much of which is otherwise destined to end up on beaches, where it may become an environmental nuisance and will decompose, returning its carbon to the atmosphere. This may also serve as a way of generating public support for macroalgae CDR given the considerable social, economic, and environmental issues caused by Sargassum patches.
- Review oceanographic data from past cruises, coastal observing systems, and buoy data to acquire needed physical, chemical, and biological information to assess site potential for field experiments.
- Data from pilot studies investigating the effects of kelp on local mitigation of ocean acidification (e.g., in the state of Washington, USA) may also provide useful information on macroalgae growth rates/carbon sequestration rates, as well as environmental co-benefits and risks.
- Gouvêa LP, Assis J, Gurgel CFD, Serrão EA, Silveira TCL, Santos R, Duarte CM, Peres LMC, Carvalho VF, Batista M, Bastos E, Sissini MN, Horta PA. Golden carbon of Sargassum forests revealed as an opportunity for climate change mitigation. Sci Total Environ. 2020 Aug 10;729:138745. doi: 10.1016/j.scitotenv.2020.138745. Epub 2020 Apr 17. Erratum in: Sci Total Environ. 2021 Apr 15;765:144696. PMID: 32498159.
Develop New In-Water Tools for Autonomous CDR Operations
A new suite of durable, seagoing technologies are needed to support macroalgae CDR RD&D. Technology development needs include:
- The recently announced Ocean and Climate Innovation Accelerator (OCIA), a partnership between the Woods Hole Oceanographic Institution and Analog Devices, Inc. aims to accelerate the development and deployment of ocean sensors and could serve as a model for accelerating sensor development in other places
Develop CDR Monitoring and Verification Protocols
Standardized methodologies from third parties to verify uptake of atmospheric CO2 resulting from macroalgae carbon sequestration will ultimately need to be developed to enable trading of carbon removal credits. Key first steps to support development of these protocols include:
- J -B E Thomas, M Sodré Ribeiro, J Potting, G Cervin, G M Nylund, J Olsson, E Albers, I Undeland, H Pavia, F Gröndahl, A comparative environmental life cycle assessment of hatchery, cultivation, and preservation of the kelp Saccharina latissima, ICES Journal of Marine Science, 2020;, fsaa112, https://doi.org/10.1093/icesjms/fsaa112
Accelerate RD&D Through New Partnerships
Research, development, and demonstration of macrolgae CDR may be accelerated and strengthened by creating partnerships with key industries/sectors, including:
- Integrated multi-trophic aquaculture1: Integration with finfish and shellfish to leverage cost savings with operations and achieve permaculture-style benefits (macroalgae can take up waste products generated by fish; fish can feed on macroalgae, the increase in pH from the presence of algae can also be beneficial for shellfish whose growth is already threatened by ocean acidification).
- Offshore wind farms are often viewed as “dead space” for other marine spatial uses, but could provide power sources and platforms for macroalgal farms
- Microalgae companies to adopt best practices for shore-based nursery facilities, such as nutrient and light needs to optimize growth and facility design(s) that maximize growth potential and minimize cost.
Developing and strengthening relationships with partner industries may also help promote public support, as well as potentially offer faster routes to obtaining the necessary permitting.
- García-Poza, S., Leandro, A., Cotas, C., Cotas, J., Marques, J.C., Pereira, L. and Gonçalves, A.M., 2020. The Evolution Road of Seaweed Aquaculture: Cultivation Technologies and the Industry 4.0. International Journal of Environmental Research and Public Health, 17(18), p.6528.
Broaden Funding Base for RD&D
Injection of significant funding is critical to move forward needed RD&D projects for CDR generally, for ocean-based CDR, and for macroalgae CDR specifically. In addition to national and subnational governmental support that is vitally needed and has been outlined in the Expanding Finance and Investment road map, additional stakeholders that need to be engaged include:
- O’Shea, T., Jones, R., Markham, A., Norell, E., Scott, J., Theuerkauf, S., and T. Waters. 2019. Towards a Blue Revolution: Catalyzing Private Investment in Sustainable Aquaculture Production Systems. The Nature Conservancy and Encourage Capital, Arlington, Virginia, USA.
- Sustainable Seaweed Solutions, www.ess.uci.edu/~sjdavis/seaweed.html.
Growing & Maintaining Public Support
First-Order Priorities to build public support for ocean-based CDR pathways are found in the Public Support road map, but there are some specific elements that can be emphasized to cultivate public support around macroalgae-based CDR:
These include:
- The perceived advantage of nature-based approaches for macroalgae pathways1
- Identification and quantification of the co-benefits associated with macroalgae cultivation
- Inclusion of coastal blue carbon ecosystems as part of a campaign to build broad public support for macroalgae.
- Bertram C and Merk C (2020) Public Perceptions of Ocean-Based Carbon Dioxide Removal: The Nature-Engineering Divide? Front. Clim. 2:594194. doi: 10.3389/fclim.2020.594194
Ocean Alkalinity Enhancement
State of Technology
Overview
This document covers technologies designed to produce ocean alkalinity enhancement (OAE) from:
- Mining and distribution of natural rock-based alkaline minerals, including olivine and other silicate rocks as well as limestone and other carbonate minerals, either in open ocean (ocean liming) or coastal environments (coastal enhanced weathering1891011.
- Production of hydroxide minerals, including lime/slaked lime2 from thermal calcination and magnesium hydroxide from synthetic weathering of olivine3 and distribution in the open ocean.
- Accelerated weathering of limestone (AWL) in ex-situ reactors to sequester non-fossil, point source CO2 emissions. Note that AWL requires a concentrated source of CO2 in seawater because carbonate minerals are oversaturated in seawater at ambient CO2 concentrations.
- Production and addition of hydrated carbonate minerals to seawater for increased alkalinity12
In contrast to recent reports456, we consider these three pathways of OAE together because of the common upstream and downstream considerations necessary to accelerate the development and testing of OAE pathways.
We consider all electrochemical-based technologies for ocean-based CDR, including alkalinity enhancement, in a separate road map due to their separate set of upstream and downstream considerations for electrochemical processes.
We also do not consider enhanced rock weathering in terrestrial ecosystems here despite its similarities with coastal enhanced weathering and its potential for gigaton-scale CDR7. Enhanced rock weathering in agricultural fields presents its own set of obstacles and development needs, many of which are distinct from those of the marine pathways considered in these road maps.
- Meysman FJR, Montserrat F. (2017) Negative CO2 emissions via enhanced silicate weathering in coastal environments. Biol. Lett. 13: 20160905. http://dx.doi.org/10.1098/rsbl.2016.0905
- Kheshgi, H. S. (1995) ‘Sequestering atmospheric carbon dioxide by increasing ocean alkalinity’, Energy, 20(9), pp. 915–922. doi: https://doi.org/10.1016/0360-5442(95)00035-F.
- Scott, A., Oze, C., Shah, V. et al. Transformation of abundant magnesium silicate minerals for enhanced CO2 sequestration. Commun Earth Environ 2, 25 (2021). https://doi.org/10.1038/s43247-021-00099-6
- Gagern, A., Rau, G. and Rodriguez, D. I. (no date) ‘Ocean Alkalinity Enhancement: Current state of knowledge and potential role of philanthropy’, p. 50.
- Energy Futures Initiative. “Uncharted Waters: Expanding the Options for Carbon Dioxide Removal in Coastal and Ocean Environments.” December 2020.
- Rackley, S.A. (2020). Ocean alkalinity enhancement: A preliminary research agenda and technology maturation roadmap. CarbonActionNow! Working paper, November 2020.
- Beerling, D.J., Kantzas, E.P., Lomas, M.R. et al. Potential for large-scale CO2 removal via enhanced rock weathering with croplands. Nature 583, 242–248 (2020). https://doi.org/10.1038/s41586-020-2448-9
- Renforth, P., and G. Henderson (2017), Assessing ocean alkalinity for carbon sequestration, Rev. Geophys., 55, 636–674, doi:10.1002/2016RG000533.
- Lenton (2018) Assessing carbon dioxide removal through global and regional ocean alkalinization under high and low emission pathways. Earth System Dynamics 9, 339-357. https://doi.org/10.5194/esd-9-339-2018.
- Köhler P, Abrams J F, Völker C, Hauck J, andWolf-Gladrow D A (2013) Geoengineering Impact of Open Ocean Dissolution of Olivine on Atmospheric CO2, Surface Ocean pH and Marine Biology. Environmental Research Letters 8(1), 014009. https://doi.org/10.1088/1748-9326/8/1/014009
- Köhler P, Hartmann J and Wolf-Gladrow D A (2010) Geoengineering potential of artificially enhanced silicate weathering of olivine. Proceedings of the National Academy of Sciences of the United States of America 107(47), 20228–20233. https://doi.org/10.1073/pnas.1000545107
- Renforth, P., Baltruschat, S., Peterson, K., Mihailova, B. D., & Hartmann, J. (2022). Using ikaite and other hydrated carbonate minerals to increase ocean alkalinity for carbon dioxide removal and environmental remediation. Joule, 6(12), 2674-2679. https://doi.org/10.1016/j.joule.2022.11.001
Technology Readiness
Addition of carbonate minerals to stabilize seawater carbonate chemistry has already been occurring in aquaculture facilities for ~10 years1, largely as a response to the hatchery failures ~2010 in the US Pacific Northwest and British Columbia from ocean acidification2. But for large scale OAE in open-ocean environments, current technological readiness is largely limited to laboratory experiments and/or modeling studies (~3-5 on a technological readiness scale3). There are now starting to be a few completed mesocosm experiments and field trials, with more planned in the future.
- The Chalk-Ex experiment released crushed calcium carbonate in a ~1.5 km2 patch in the Gulf of Maine, although the primary objective of this experiment was to quantify the effects of particles on upper ocean layer optical properties, not to achieve CDR4.
- There has been at least one small-scale (~50m x 50m) OAE field trial (sodium hydroxide addition) on a coral atoll in the Great Barrier Reef in order to evaluate the ecosystem responses to ocean acidification mitigation5.
- Vesta has a demonstration pilot of coastal enhanced weathering of olivine in Southampton, NY9, adding 500 cubic yards of olivine sand to the second phase of a beach nourishment effort. 6
- Researchers at the Universiteit Antwerpen and Delft University of Technology are planning a 1000 ton coastal enhanced weathering field experiment in the North Sea in 20247.
- Mesocosm experiments have been conducted as part of the OceanNETs project in the European Union’s Horizon 2020 program8.
- Giri, Bruno J., and Claude E. Boyd. “Effects of Frequent, Small Doses of Calcium Carbonate on Water Quality and Phytoplankton in Channel Catfish Ponds.” North American Journal of Aquaculture 62, no. 3 (2000): 225–28. http://dx.doi.org/10.1577/1548-8454(2000)062%3C0225:EOFSDO%3E2.3.CO;2
- Washington State Blue Ribbon Panel on Ocean Acidification (2012): Ocean Acidification: From Knowledge to Action, Washington State’s Strategic Response. H. Adelsman and L. Whitely Binder (eds). Washington Department of Ecology, Olympia, Washington. Publication no. 12-01-015.
- TRL definitions: https://www.nasa.gov/directorates/heo/scan/engineering/technology/txt_accordion1.html
- Balch, W. M., Plueddeman, A. J., Bowler, B. C., and Drapeau, D. T. (2009), Chalk‐Ex—Fate of CaCO3 particles in the mixed layer: Evolution of patch optical properties, J. Geophys. Res., 114, C07020, doi:10.1029/2008JC004902.
- Albright, R., Caldeira, L., Hosfelt, J. et al. Reversal of ocean acidification enhances net coral reef calcification. Nature 531, 362–365 (2016). https://doi.org/10.1038/nature17155
- Vesta / The Plan, https://www.projectvesta.org/plan#Phase-1.
- Comments from Dr. Filip Meysman, ‘Workshop on Ocean-based CDR Opportunities and Challenges, Part 1: Setting the Stage’, A Research Strategy for Ocean Carbon Dioxide Removal and Sequestration, U.S. National Academy of Sciences. 19th January 2021. Accessible at: https://www.nationalacademies.org/event/01-19-2021/a-research-strategy-for-ocean-carbon-dioxide-removal-and-sequestration-workshop-series-part-1
- “About the Project.” OceanNETs, https://www.oceannets.eu/about-the-project/.
- https://www.vesta.earth/southampton
CDR Potential
- Carbon Capture
Due to the vast quantities of alkaline rocks, and the capacity of the ocean to accommodate similarly vast quantities of bicarbonate ions, OAE has the theoretical potential to capture tens of gigatons of carbon dioxide annually1. But given current technological readiness, this remains theoretical. Recent consensus reports cite the following as possible carbon dioxide removal potential from ocean alkalinity enhancement:
- >0.1 – 1.0 Gt CO2/year (NASEM 2022: A Research Strategy for Ocean-Based Carbon Dioxide Removal and Sequestration)
- 1 – 15+ Gt CO2/ year (NOAA 2022: Carbon Dioxide Removal Research)
Technical, economic, social, political, and governance factors may also decrease this theoretical CDR potential, although the degree to which they limit the CDR remains to be determined. Cost estimates currently vary between studies with estimated ranges of $72-159/ton CO2 (variation due to variations in source rock, means of mining and production2}), $60-110/ton CO23, and $<25-125/ton CO24. More work is necessary to produce ground-truthed cost estimates.
- Sequestration Permanence
OAE will result in additional CO2 from the atmosphere being sequestered in the ocean as bicarbonate ions, which cannot exchange with the atmosphere. The residence time of bicarbonate ions in the ocean is ~10,000 years, suggesting that OAE sequestration would be stable for ~10,000 years.
- Accelerated Weathering of Limestone: Avoided Emissions and Hybrid Methods
When AWL is used to sequester carbon dioxide resulting from the combustion of fossil fuels, it represents avoided emissions, not removal of atmospheric carbon dioxide (negative emissions)56. However, there exist a number of hybrid possibilities to integrate AWL with various non-fossil sources of concentrated carbon dioxide to permanently sequester the carbon dioxide as bicarbonate ions in the ocean. These hybrid approaches include:
- AWL coupled with direct air capture – captured CO2 from a direct air capture (DAC) facility can be concentrated and reacted with limestone in the presence of seawater to trap the captured CO2 as bicarbonate ions.
- The bicarbonate-rich effluent of DAC + AWL can also serve as a substrate to support algal growth78; however newly-produced algal carbon must be sequestered from return to the atmosphere to generate negative emissions over relevant permanence timescales (> 100 years).
- AWL coupled with bioenergy – CO2 generated from the combustion of biomass (either terrestrial or marine-based) to generate energy can be concentrated and reacted with limestone in the presence of seawater to trap the CO2 as bicarbonate ions. This process is analogous to bioenergy with carbon capture and storage (BECCS)9, except that the carbon is stored as bicarbonate ions in the ocean as opposed to being sequestered in geologic reservoirs.
We further consider AWL in these hybrid configurations that generate the removal of atmospheric carbon dioxide and sequestration as bicarbonate in the ocean.
- AWL coupled with direct air capture – captured CO2 from a direct air capture (DAC) facility can be concentrated and reacted with limestone in the presence of seawater to trap the captured CO2 as bicarbonate ions.
- Gagern, Antonius. “Ocean Alkalinity Enhancement: Current state of knowledge and potential role of philanthropy”. 9 September 2019. Meeting Proceedings Half Moon Bay, California.
- Renforth, P., and G. Henderson (2017), Assessing ocean alkalinity for carbon sequestration, Rev. Geophys., 55, 636–674, doi:10.1002/2016RG000533.
- Gattuso J-P, Williamson P, Duarte CM and Magnan AK (2021) The Potential for Ocean-Based Climate Action: Negative Emissions Technologies and Beyond. Front. Clim. 2:575716. doi: 10.3389/fclim.2020.575716
- EFI Report “Uncharted Waters: Expanding the Options for Carbon Dioxide Removal in Coastal and Ocean Environments.” December 2020.
- Rau, G. H. and Caldeira, K. (1999) ‘Enhanced carbonate dissolution: a means of sequestering waste CO2 as ocean bicarbonate’, Energy Conversion, p. 11.
- Rau, G. H. (2011) ‘CO 2 Mitigation via Capture and Chemical Conversion in Seawater’, Environmental Science & Technology, 45(3), pp. 1088–1092. doi: 10.1021/es102671x.
- Rau, G.H. (2014). Use of Carbonates for Biological and Chemical Synthesis. (U.S. Patent No. 8,828,706 B2). https://patentimages.storage.googleapis.com/f0/75/e4/4dd652a83ecd1c/US8828706.pdf
- “Development of High Value Bioproducts and Enhancement of Direct-Air Capture Efficiency with a Marine Algae Biofuel Production System,” n.d., 2.
- Hughes, Adam D., et al. “Does Seaweed Offer a Solution for Bioenergy with Biological Carbon Capture and Storage?” Greenhouse Gases: Science and Technology, vol. 2, no. 6, 2012, pp. 402–407., doi:10.1002/ghg.1319.
Environmental Co-benefits
- OAE would likely provide localized reductions in ocean acidification, with expected benefit(s) to marine ecosystems12.
- In certain areas, calcium or silica additions could act as fertilizers to support plankton populations3.
- Gattuso J-P, Magnan AK, Bopp L, Cheung WWL, Duarte CM, Hinkel J, Mcleod E, Micheli F, Oschlies A, Williamson P, Billé R, Chalastani VI, Gates RD, Irisson J-O, Middelburg JJ, Pörtner H-O and Rau GH (2018) Ocean Solutions to Address Climate Change and Its Effects on Marine Ecosystems. Front. Mar. Sci. 5:337. doi: 10.3389/fmars.2018.00337
- Feng (冯玉铭), Ellias Y, David P Keller, Wolfgang Koeve, and Andreas Oschlies. “Could Artificial Ocean Alkalinization Protect Tropical Coral Ecosystems from Ocean Acidification?” Environmental Research Letters 11, no. 7 (July 1, 2016): 074008. https://doi.org/10.1088/1748-9326/11/7/074008.
- Adhiya, Jagat, and Sallie W Chisholm. “Is Ocean Fertilization a Good Carbon Sequestration Option?,” Massachusetts Institute of Technology Laboratory for Energy and the Environment n.d., 70.
Environmental Risks
- OAE may pose ecotoxicological risks from the release of elevated concentrations of trace metals from some minerals during mineral dissolution1.
- The toxicity will depend on the source rock, the concentration of source rock applied, and the seawater chemistry (which determines bioavailability). These trace metal additions could also pose risks to human health if accumulation occurs through food webs2.
- Silicate rocks are likely to have higher metal concentrations than carbonate rocks, and thus may pose a greater ecotoxicological risk2
- In-situ applications of alkaline materials (e.g. coastal enhanced weathering, ocean liming) may pose greater ecotoxicological risks than ex-situ applications (e.g. reactors for accelerated weathering of limestone) because of the ability to capture and treat the effluent from ex-situ reactors before release into the ocean. However, options exist to mitigate these effects e.g., through rapid dilution in the wake of vessels3.
- The potential for changes in water column particle concentrations, turbidity, and optical properties from dispersing fine particulates
- The potential for changes in seafloor deposition of particles and their effects on smothering or burial, food webs interactions, light availability and more
- Large-scale mining of silicate or carbonate rocks may pose environmental risks, similar to those typically associated with existing mines including ground vibrations from blasting, noise pollution, decreased air and soil quality, etc.
- Ecological and geochemical impacts of discharging high alkalinity/high pH waters, including precipitation (inorganic mineral formation) of carbonates. However, options to mitigate these effects exist e.g., through rapid dilution in ship's wakes3.
- OAE may have the potential to shift phytoplankton community composition from carbonate-shell producing plankton (coccolithophores) to silica-shell producing plankton (diatoms) or vice-versa depending on the form of alkalinity addition.
- Potential for bioaccumulation and biomagnification in the food chain4
- Dedicated ships/vessels to distribute minerals could cause environmental impacts such as additional noise pollution5, the potential transfer of nonindigenous species 6, and the addition of pollutants from shipping emissions7.
- “Antacids for the Sea? Artificial Ocean Alkalinization and Climate Change | Elsevier Enhanced Reader.” Accessed March 17, 2021. https://doi.org/10.1016/j.oneear.2020.07.016.
- Bach LT, Gill SJ, Rickaby REM, Gore S and Renforth P (2019) CO2 Removal With Enhanced Weathering and Ocean Alkalinity Enhancement: Potential Risks and Co-benefits for Marine Pelagic Ecosystems. Front. Clim. 1:7. doi: 10.3389/fclim.2019.00007
- Caserini S, Pagano D, Campo F, Abbà A, De Marco S, Righi D, Renforth P and Grosso M (2021) Potential of Maritime Transport for Ocean Liming and Atmospheric CO2 Removal. Frontiers in Climate 3, 22. https://doi.org/10.3389/fclim.2021.575900.
- National Academies of Sciences, Engineering, and Medicine 2022. A Research Strategy for Ocean-based Carbon Dioxide Removal and Sequestration. Washington, DC: The National Academies Press. https://doi.org/10.17226/26278.
- Kaplan, M. B., & Solomon, S. (2016). A coming boom in commercial shipping? The potential for rapid growth of noise from commercial ships by 2030. Marine Policy, 73, 119-121. https://doi.org/10.1016/j.marpol.2016.07.024
- Muirhead, Jim R., et al. “Projected Effects of the Panama Canal Expansion on Shipping Traffic and Biological Invasions.” Diversity and Distributions, vol. 21, no. 1, 2014, pp. 75–87., https://doi.org/10.1111/ddi.12260.
- Dalsøren, S. B., Samset, B. H., Myhre, G., Corbett, J. J., Minjares, R., Lack, D., and Fuglestvedt, J. S.: Environmental impacts of shipping in 2030 with a particular focus on the Arctic region, Atmos. Chem. Phys., 13, 1941–1955, https://doi.org/10.5194/acp-13-1941-2013, 2013.
Social Risks
- Risks associated with the expansion on mining activities for OAE
- Demographic changes including, but not limited to, shifts in gender balance, increase in non-resident workforces, appropriation of land from local communities, social inequality, and impacts on Indigenous communities3
- Negative health impacts including cancer, respiratory diseases, injuries, and prolonged exposure to chemical agents4
- Environmental implications include impacts to soil and air quality, impacts to ground and surface waters, and erosion.
- “Antacids for the Sea? Artificial Ocean Alkalinization and Climate Change | Elsevier Enhanced Reader.” Accessed March 17, 2021. https://doi.org/10.1016/j.oneear.2020.07.016.
- Bach LT, Gill SJ, Rickaby REM, Gore S and Renforth P (2019) CO2 Removal With Enhanced Weathering and Ocean Alkalinity Enhancement: Potential Risks and Co-benefits for Marine Pelagic Ecosystems. Front. Clim. 1:7. doi: 10.3389/fclim.2019.00007
- National Academies of Sciences, Engineering, and Medicine 2022. A Research Strategy for Ocean-based Carbon Dioxide Removal and Sequestration. Washington, DC: The National Academies Press. https://doi.org/10.17226/26278.
- Candeias, C., P. Ávila, P. Coelho, and J. P. Teixeira. 2019. Mining activities: Health impacts. Pp. 415-435 in Encyclopedia of Environmental Health, 2nd ed., J. O. Nriagu, ed. Cambridge, MA: Elsevier.
Social Co-benefits
- Creation of job opportunities
- “Antacids for the Sea? Artificial Ocean Alkalinization and Climate Change | Elsevier Enhanced Reader.” Accessed March 17, 2021. https://doi.org/10.1016/j.oneear.2020.07.016.
- Bach LT, Gill SJ, Rickaby REM, Gore S and Renforth P (2019) CO2 Removal With Enhanced Weathering and Ocean Alkalinity Enhancement: Potential Risks and Co-benefits for Marine Pelagic Ecosystems. Front. Clim. 1:7. doi: 10.3389/fclim.2019.00007
Development Gaps and Needs
Addressing Knowledge Gaps
- Because of the lack of proof-of-concept field experiments, it has so far been impossible to characterize benefits, risks, and scaling consideration of OAE in real-world settings (i.e. not benchtop). Controlled field experiments across diverse ecosystems to determine marine chemistry and biology impacts and feedbacks are needed (Develop New Modeling Tools to Support Design and Evaluation, Accelerate Design and Permitting of Controlled Field Trials).
- It is challenging to verify additional CO2 uptake from the atmosphere as a result of OAE given the ocean’s dynamic CO2 flux “background state”. New methodologies are needed to observe additional sequestration from the atmosphere into the ocean (Develop New Modeling Tools to Support Design and Evaluation, Develop New In-Water Tools for Autonomous CDR Operations).
- Laboratory experiments are needed across a range of seawater chemistries expected as a result of equilibrated OAE scenarios (e.g. high total alkalinity and high dissolved inorganic carbon1), along with various alkaline materials and their associated major (e.g. magnesium, calcium) and minor (e.g. nickel, cadmium) elemental concentrations to characterize environmental impacts (Accelerate Design and Permitting of Controlled Field Trials).
- Look to the ocean acidification community’s effort to develop standardized protocols for guidance2 as the OAE community builds out standardized protocols and treatments levels for consistency and intercomparability
- Existing database(s) of ecotoxicological tests need to be reviewed3 for possible OAE source materials (e.g. Ca(OH)2) and co-occurring metals (e.g. Ni, Cd) to identify known ecotoxicological effect and lethality thresholds and current gaps in our understanding.
- Global, local, and regional predictions of physical, chemical, and biological outcomes and feedbacks of OAE from high-resolution models are needed (Develop New Modeling Tools to Support Design and Evaluation)
- Laboratory experiments are needed on silicate and carbonate mineral kinetics and dissolution catalysts4 to better understand mineral dissolution rates in marine environments and means to accelerate them (Accelerate Design and Permitting of Controlled Field Trials).
- The coastal enhanced weathering facility jointly administered by Universiteit Antwerpen, the University of Gent, and VLIZ provides mesocosm and monitoring equipment to conduct mineral dissolution experiments in tank conditions closely approximating real-world beach and shallow water settings.
- Life cycle assessments are necessary to calculate net CDR benefits, taking into account all emissions associated with supply chains (Develop CDR Monitoring and Verification Protocols).
- There may be good value in summarizing best practices, lessons learned, and pitfalls from lime treatment of acid rain-affected lakes and watersheds to accelerate OAE development and testing567
- In order to address several of the knowledge gaps listed above, Additional Ventures is partnering with Ocean Visions and a consortium of philanthropic funders (2022) to identify and support the proposal that best articulates the highest priority questions facing the testing and development of OAE approaches.
- Comments from Dr. Ros Rickaby, ‘Workshop on Ocean-based CDR Opportunities and Challenges, Part 2: Technological and Natural Approaches to Ocean Alkalinity Enhancement and CO2 Removal’ on 27th January 2021
- Gattuso, Jean-Pierre, and Lina Hansson. “European Project on Ocean Acidification (EPOCA): Objectives, Products, and Scientific Highlights.” Oceanography 22, no. 4 (December 1, 2009): 190–201. https://doi.org/10.5670/oceanog.2009.108.
- EPA, Environmental Protection Agency, https://cfpub.epa.gov/ecotox/.
- Mechanisms of calcite dissolution in seawater Adam V. Subhas, Jess F. Adkins, Nick E. Rollins, John Naviaux, Jonathan Erez, William M. Berelson Proceedings of the National Academy of Sciences Aug 2017, 114 (31) 8175-8180; DOI: 10.1073/pnas.1703604114
- Comments from Dr. Tim Kruger, ‘Workshop on Ocean-based CDR Opportunities and Challenges, Part 2: Technological and Natural Approaches to Ocean Alkalinity Enhancement and CO2 Removal’. A Research Strategy for Ocean Carbon Dioxide Removal and Sequestration, U.S. National Academy of Sciences. 27th January 2021. Accessible at: https://www.nationalacademies.org/event/01-27-2021/a-research-strategy-for-ocean-carbon-dioxide-removal-and-sequestration-workshop-series-part-2
- Taylor, L. L. et al. (2021) ‘Increased carbon capture by a silicate-treated forested watershed affected by acid deposition’, Biogeosciences, 18(1), pp. 169–188. doi: 10.5194/bg-18-169-2021.
- Naturresursavdelningen (2010) Kalkning av sjöar och vattendrag. Handbok 2010:2. 92pp Accessible at: https://www.naturvardsverket.se/Documents/publikationer/978-91-620-0165-0.pdf
Engineering Challenges and Needs
- Current observational technologies (sensors, ROVs, AUVs, etc.) and modeling tools are not widespread and easily available to fully support field trials with the desired spatial and temporal frequency of monitoring and sampling needed (Develop New In-Water Tools for Autonomous CDR Operations).
- New technologies are needed to reduce the cost and environmental impacts of mining, grinding, and distribution of alkaline rocks with minimum environmental impact1.
- Assessment of the potential to re-purpose existing supply chains for coal and cement to provide an easier ramp up to meet the production and distribution scales required (Accelerate RD&D Through New Partnerships).
- Assessment of the potential to scale the cement and lime industries to meet the expected needs of calcination23(Accelerate RD&D Through New Partnerships).
- Identification of sources of renewable energy at sufficient scale to power OAE (Develop New In-Water Tools for Autonomous CDR Operations)
- For in-situ application with hydroxide minerals, methods to handle the heat generated from adding hydroxides to the ocean because this is an exothermic (heat-releasing) reaction (Develop New In-Water Tools for Autonomous CDR Operations).
- For point source OAE, cost-effective and safe methods need to be developed that optimize the distribution, dispersal and dilution of any strong chemical bases to avoid impacts of excessively alkaline (pH>9) waters on marine ecosystems. Dilution may require pumping large amounts of seawater, which need to be optimized for energy and cost (Develop New In-Water Tools for Autonomous CDR Operations). However, it may be possible to use existing large-volume discharges such as power station cooling water discharges that can be as high as 90 cubic meters per second for a 1600 MWe nuclear power station. In addition, another option would be to utilize the rapid dilution available in ships' wakes4.
- See this relevant example of a recent analysis of the energy costs associated with mineral grinding for enhanced rock weathering in terrestrial environments; Beerling, D.J., Kantzas, E.P., Lomas, M.R. et al. Potential for large-scale CO2 removal via enhanced rock weathering with croplands. Nature 583, 242–248 (2020). https://doi.org/10.1038/s41586-020-2448-9
- Renforth, P., B. G. Jenkins, and T. Kruger. 2013. “Engineering Challenges of Ocean Liming.” Energy 60: 442–52.
- Renforth, P., and G. Henderson (2017), Assessing ocean alkalinity for carbon sequestration, Rev. Geophys., 55, 636–674, doi:10.1002/2016RG000533
- Caserini S, Pagano D, Campo F, Abbà A, De Marco S, Righi D, Renforth P and Grosso M (2021) Potential of Maritime Transport for Ocean Liming and Atmospheric CO2 Removal. Frontiers in Climate 3, 22. https://doi.org/10.3389/fclim.2021.575900.
Building an OAE Market
- Development of a robust carbon market for OAE-derived CDR is especially important because most OAE pathways as described above do not produce co-products that could generate revenue and lower overall system costs.
- However, synthetic production of magnesium hydroxide via accelerated weathering of olivine co-produces silica and metal oxides1, both of which can be sold as co-products to reduce the overall system costs
- Strengthening mechanisms to protect intellectual property associated with various OAE pathways need to be developed.
- Development of third-party verification protocols is needed to support trading of carbon removal credits on carbon markets (Develop CDR Monitoring and Verification Protocols)
- Scott, A., Oze, C., Shah, V. et al. Transformation of abundant magnesium silicate minerals for enhanced CO2 sequestration. Commun Earth Environ 2, 25 (2021). https://doi.org/10.1038/s43247-021-00099-6
Public Awareness and Support Are Low
Many of the opportunities and challenges around building public awareness and support for ocean-based CDR are not specific to OAE, but there are several hurdles specific to OAE:
- OAE faces challenges in terms of public perception of potential environmental risks that are not necessarily faced by what are considered more “nature-based” approaches (e.g., coastal blue carbon restoration)1 (Growing and Maintaining Public Support).
- There is a great deal of hesitancy and resistance around any ocean-based CDR pathway that relies on adding materials to the ocean.
- Earlier ocean iron fertilization experiments2 could offer opportunities to adopt best practices and avoid mistakes made when building public support for OAE.
- Negative perceptions exist about the large-scale mining that would likely be needed to generate sufficient rock supplies to support global-scale OAE (Growing and Maintaining Public Support).
- Clearer communication strategies need to be developed to respond to the “geoengineering” narrative of OAE (Growing and Maintaining Public Support).
- Bertram C and Merk C (2020) Public Perceptions of Ocean-Based Carbon Dioxide Removal: The Nature-Engineering Divide? Front. Clim. 2:594194. doi: 10.3389/fclim.2020.594194
- Schiermeier, Q. (2009a). Ocean Fertilization Experiment Draws Fire: Indo-German Research Cruise Sets Sail Despite Criticism. Available online at: https://www.nature.com/news/2009/090109/full/news.2009.13.html
Filling Governance Gaps
Advancing the development and testing of OAE will require governance structures that both enable the permitting of legitimate testing and development and ensure that the public interests are protected.
- There is currently no clear international regime that governs research and development in OAE. The closest regimes would be the London Convention and the London Protocol, but currently they are not built to govern OAE scientific field trials in marine waters1.
- Small-scale OAE field trials by “invited Parties and other Governments” with prior environmental impact assessments may be allowed under the United Nations Convention on Biological Diversity (CBD) Section X/33(8)(w). The CBD, however, is not legally binding and not all countries are party to the CBD (for example, the United States)
- Note that the London Convention and London Protocol cover all waters up to the baselines used to measure the territorial sea and the EEZ. In addition, London Protocol Parties have to either apply the Protocol to marine internal waters (i.e., behind the baselines e.g., estuaries) or they have to adopt other effective permitting and regulatory measures for marine internal waters.
First-Order Priorities
Develop New Modeling Tools to Support Design and Evaluation
High-resolution data-assimilative models are needed to support real-world testing of OAE. These modeling tools must:
- Account for complex interactions in the immediate vicinity of the alkalinity release and downstream impacts
- Provide four-dimensional (space and time) estimates of biogeochemistry in zone of influence both in the presence and absence of OAE. The difference between these two simulations can be used to inform CDR estimates that account for background variability in the ocean.
- CDR estimates from OAE must include estimates of the “opportunity cost” of OAE - how did OAE shift phytoplankton community composition, production, and export?
To support the design of proof-of-concept field trials, these models should also:
- Provide estimates of the size and scale of biogeochemical modification to the ecosystem from OAE, allowing for informed placement of sensors to monitor the field trials
- Be capable of simulating passive tracers (e.g. SF6) to inform whether and how these passive tracers may be useful in field trials (e.g., estimating rates of atmospheric CO2 uptake)
- Inform a prioritized set of predictions to be tested during field trials
Accelerate Design and Permitting of Controlled Field Trials
Field trials for the various OAE technologies are urgently needed to test both carbon sequestration potential and environmental impacts (both positive and negative). A series of activities and products are needed to get to a series of controlled field trials. They include:
- Albright, R., Caldeira, L., Hosfelt, J. et al. Reversal of ocean acidification enhances net coral reef calcification. Nature 531, 362–365 (2016). https://doi.org/10.1038/nature17155
Develop New In-Water Tools for Autonomous CDR Operations
A new suite of durable, seagoing technologies are needed to support OAE RD&D. Technology development needs include:
- MEPC (1975) Method for calculation of dilution capacity in the ships wake. Submitted jointly by the Netherlands and Norway
- “MAMPEC.” Deltares, 31 Jan. 2017, www.deltares.nl/en/software/mampec/.
- Caserini, S., Pagano, D., Campo, F., Abbà, A., De Marco, S., Righi, D., Renforth, P. and Grosso, M., 2021. Potential of Maritime Transport for Ocean Liming and Atmospheric CO2 Removal. Frontiers in Climate, 3, p.22. https://doi.org/10.3389/fclim.2021.575900
Develop CDR Monitoring and Verification Protocols
Standardized methodologies from third parties to verify uptake of atmospheric CO2 resulting from ocean alkalinity enhancement will ultimately need to be developed to enable trading of carbon removal credits. Key first steps to support development of these protocols include:
- Convening experts to review advances from modeling tools (Develop New Modeling Tools to Support Design and Evaluation) and controlled field trials (Accelerate Design and Permitting of Controlled Field Trials) to identify satisfied and outstanding data needs necessary to quantify additional CO2 uptake as a direct result of OAE. As advances in OAE RD&D are made, the satisfied and outstanding data needs will need to be updated.
- Apply existing12 or develop when necessary, life cycle analysis tools to calculate stored carbon after accounting for emissions from required materials, energy, transportation/dispersal, etc.
- Include aspects of sustained monitoring to verify CDR permanence over long time scales as CDR is scaled.
- Koornneed, J. and Nieuwlaar, E., 2009. Environmental life cycle assessment of CO2 sequestration through enhanced weathering of olivine. Working paper, Group Science, Technology and Society, Utrecht University.
- Hartmann, J., West, A.J., Renforth, P., Köhler, P., De La Rocha, C.L., Wolf‐Gladrow, D.A., Dürr, H.H. and Scheffran, J., 2013. Enhanced chemical weathering as a geoengineering strategy to reduce atmospheric carbon dioxide, supply nutrients, and mitigate ocean acidification. Reviews of Geophysics, 51(2), pp.113-149.
Accelerate RD&D Through New Partnerships
Research, development, and demonstration of OAE may be accelerated and strengthened by creating partnerships with key industries/sectors, including:
- Transoceanic shipping – cargo vessels often travel at less than full capacity, provide onboard power, and move from port to port providing frequent opportunities to acquire new alkaline material and offload byproducts from alkalinization processes. Developing partnerships with the shipping industry to help the industry fulfill its commitments to decarbonize shipping could be a stepping stone to accelerate ocean-based net negative emissions (e.g. Poseidon Principles1).
- Industries generating alkaline byproducts for feedstock into OAE processes (steel, aluminum, cement, lime, and nickel production; coal and biomass combustion)2.
- 7 billion tons of alkaline byproducts generated annually from manufacturing and combustion could decrease costs and potentially facilitate transitions to larger-scale OAE3.
- Finfish and shellfish aquaculture, as well as coral reefs, where the added alkalinity could be used to optimize chemical conditions, including providing relief from ocean acidification4.
- Offshore renewable energy production, including wind and others, both as power sources and as integrated CDR platforms.
- Coastal industries, including desalination and wastewater treatment facilities, which already have infrastructure for pumping/processing seawater or wastewater for alkalinity addition.
- Marine research laboratories that already pump seawater and have expertise, technical equipment and infrastructure to support research and development.
Developing and strengthening relationships with partner industries may also help promote greater public support, as well as potentially offer faster routes to obtaining the necessary permitting.
- “Poseidon Principles” https://www.poseidonprinciples.org/
- Renforth, P. The negative emission potential of alkaline materials. Nat Commun 10, 1401 (2019). https://doi.org/10.1038/s41467-019-09475-5
- Renforth, P. The negative emission potential of alkaline materials. Nat Commun 10, 1401 (2019). https://doi.org/10.1038/s41467-019-09475-5
- Gattuso J-P, Magnan AK, Bopp L, Cheung WWL, Duarte CM, Hinkel J, Mcleod E, Micheli F, Oschlies A, Williamson P, Billé R, Chalastani VI, Gates RD, Irisson J-O, Middelburg JJ, Pörtner H-O and Rau GH (2018) Ocean Solutions to Address Climate Change and Its Effects on Marine Ecosystems. Front. Mar. Sci. 5:337. doi: 10.3389/fmars.2018.00337
Growing and Maintaining Public Support for Research and Development
First-Order Priorities to build public support for ocean-based CDR pathways are found in the Public Support road map Additionally, there are some specific opportunities to cultivate public engagement in and support around OAE including:
- Developing targeted public outreach/advocacy campaigns to inform about OAE and its potentials with regards to ameliorating ocean acidification
- Responding to the narrative of OAE as less “nature-based” than, for instance, coastal blue carbon restoration with the concept that OAE represents an acceleration of “natural” chemical weathering.
Microalgae Cultivation and Carbon Sequestration
State of Technology
Overview
- Open systems where the open ocean is directly manipulated to enhance biological production, capture atmospheric CO2, and export the captured carbon to the deep ocean. In open systems, CO2 fixation is facilitated by the addition of limiting macronutrients (e.g., phosphorus, nitrogen, silica) and/or micronutrients (e.g., iron) to the ocean’s surface to augment biological production 2. Open system techniques accelerate natural processes already occurring in the ocean. Most approaches in the open ocean fall into the following two categories (some proposals that do not fit into these categories are also explored).
- Surface nutrient addition: the direct addition of nutrients (macro or micro) into ocean waters in situ to increase microalgal growth
- Nutrient upwelling: artificial upwelling of nutrient-rich deep ocean waters to the surface to increase microalgal growth
- Closed systems where inputs and growth conditions are controlled, and outputs (microalgae) are harvested within the confines of a pond or a photobioreactor. In closed systems, CO2 fixation is facilitated by the mixing of required inputs (sunlight, nutrients, CO2, water) and the introduction of microalgae culture with the intention of reproduction and continuous fixation in a contained system 3. This can be accomplished on shore in cultivation ponds or photobioreactors, or afloat in photobioreactors either stationary or towed at sea 456.
-
- Onshore: encompasses more established methods of microalgae cultivation, including photobioreactors, cultivation ponds, and hybrid onshore configurations. In these systems, all inputs are tightly controlled and regulated, and outputs must be directly managed through either storage or utilization of byproducts. While cultivation techniques are well-established and show high technological readiness, storage and utilization pathways remain underdeveloped and scale is a major consideration. Social and environmental risks for closed onshore systems are easier to monitor and mitigate due to the controlled nature of the system.
- Offshore: includes floating photobioreactors (PBRs) that are incorporated into a floating platform which can be stationary or towed behind a ship. In these systems, cultivation occurs in the photobioreactor, inputs are regulated, and outputs can be actively managed or directed. While at sea, these photobioreactors operate much like their onshore counterparts to cultivate microalgae, however nutrients and energy are provided by the ocean water and wave action, respectively. After microalgae are cultivated, they can be sunk into the deep ocean for sequestration or hauled to shore to be used as biomass. This is an area that has garnered much attention in startup communities (see this Y Combinator request for startups), however, little is available about the technologies in the open-sourced or peer reviewed literature.
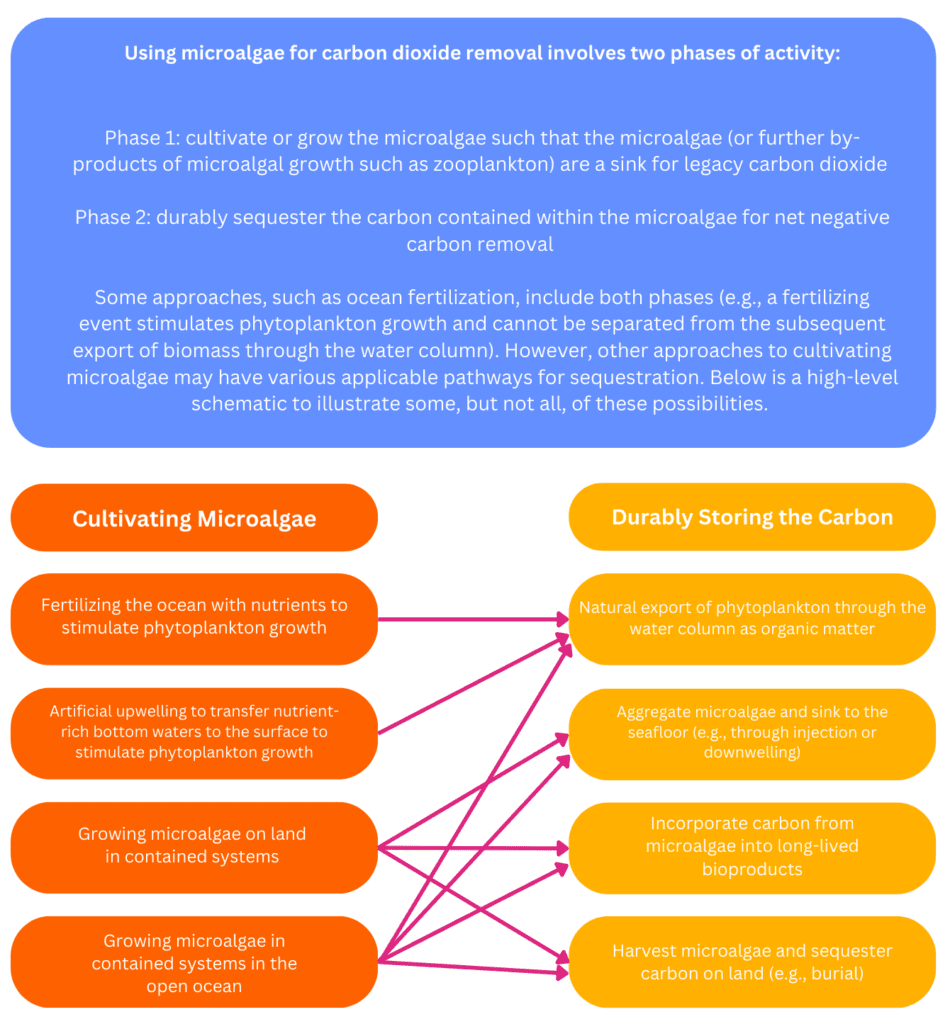
- Bhola, V., et al. “Overview of the Potential of Microalgae for CO2 Sequestration.” International Journal of Environmental Science and Technology, vol. 11, no. 7, 2014, pp. 2103–2118., https://doi.org/10.1007/s13762-013-0487-6.
- Williamson, Phillip, et al. “Ocean Fertilization for Geoengineering: A Review of Effectiveness, Environmental Impacts and Emerging Governance.” Process Safety and Environmental Protection, vol. 90, no. 6, Nov. 2012, pp. 475–88, https://doi.org/10.1016/j.psep.2012.10.007.
- Beal, C., et al. “Marine microalgae commercial production improves sustainability of global fisheries and aquaculture.” Scientific reports, vol. 8, no.1, 2018b, pp. 1-8.
- Zhai, Xiaoqian, et al. “Seawater Supplemented with Bicarbonate for Efficient Marine Microalgae Production in Floating Photobioreactor on Ocean: A Case Study of Chlorella Sp.” Science of The Total Environment, vol. 738, Oct. 2020, p. 139439, https://doi.org/10.1016/j.scitotenv.2020.139439.
- Kim, Z.-Hun, et al. “Development of a Floating Photobioreactor with Internal Partitions for Efficient Utilization of Ocean Wave into Improved Mass Transfer and Algal Culture Mixing.” Bioprocess and Biosystems Engineering, vol. 39, no. 5, May 2016, pp. 713–23, https://doi.org/10.1007/s00449-016-1552-6.
- Zhu, Chenba, et al. “Bicarbonate-Based Carbon Capture and Algal Production System on Ocean with Floating Inflatable-Membrane Photobioreactor.” Journal of Applied Phycology, vol. 30, no. 2, Apr. 2018, pp. 875–85, https://doi.org/10.1007/s10811-017-1285-1.
Considerations for CDR Potential
Regardless of approach or technology, there are two fundamental questions that make up the “CDR Potential” for a given pathway:
1) How much carbon can this approach sequester? 2) How long can it stay sequestered?
How much carbon can be sequestered?
- Carbon fixation rates and storage capacity of the microalgae species
- The capacity of microalgae to fix carbon differs across species and selecting appropriate species for different approaches remains an area of study. Genetic modification of carbon fixation rates and carbon storage capacity may expand the CDR potential for microalgae 6.
- Scalability of the approach (for example, offshore cultivation has fewer spatial imitations than onshore cultivation)
- The expense of acquiring, transporting, and delivering nutrients, as well as the bioavailability of those nutrients, and the resulting carbon-to-nutrient ratio in the microalgae
- Additional considerations for closed systems include:
- Harvesting Considerations: The energy necessary for harvesting algae from cultivation set-ups (e.g., raceway ponds) can be an impediment to scaling. Current estimates from the US Department of Energy cite energy usage as ~1.4 kWh/m3, and high capital costs, ~$152,000/million gallons per day. Automated harvesters, such as the Zobi Harvester, have the potential to cut time and costs significantly, using less than 0.14 kwh/m3 and a capital cost of less than $106,000 per million gallons per day of capacity, unlocking harvesting barriers 1. Utilizing gravity-based systems is another way of increasing energy efficiency for harvesting 2.
- Planktonic Cultivation vs Biofilms: Microalgae can be cultivated in two forms: planktonic or on biofilms. Planktonic microalgae are suspended in water and are highly diluted (less than 1%). Planktonic microalgae have been more extensively studied and can be cultivated in photobioreactors or ponds 3. Biofilms are aggregates of microorganisms attached to a surface and are much more concentrated than planktonic cultivation. The areal productivity for biofilms of microalgae is about two-fold the one for planktonic microalgae 4. New techniques with biofilms are in development 5.
How durable is the carbon sequestration?
- Deep ocean: wherein microalgae, and their embedded carbon, cultivated via ocean fertilization or on land in photobioreactors or ponds are stored in the ocean for long-term storage. If purposely placed or injected into the water column at depth (>1,000 meters), many parts of the global ocean can offer 100 to 1,000 years-scale permanence. Sequestration durability is reduced to 10 to 100 years-scale for microalgae participating in the biological carbon pump due to high rates of remineralization in the upper ocean and during transit to the deep ocean as microalgae are grazed or decompose. There is extensive basin-scale variability in sequestration durability 7. Areas of the ocean best suited to long carbon storage are places where surface waters and sinking matter can reach depths greater than 1,000 meters. The Southern Ocean south of the biogeochemical divide separating the Antarctic from the sub-Antarctic would be one such suitable location as well as much of the North Pacific 8. Findings also suggest the North Atlantic and North Pacific oceans have the greatest carbon storage potential, while subtropical oceans have the least 9. Continued thorough measurements and models are needed to quantify the durability of sequestration for any given site, accounting for the fact that durability is likely best defined as a probability distribution given the various transit times of water masses.
-
-
- Investigating Carbon Storage in Microalgae Polysaccharides: Scientists at the MARUM MPG Bridge Group Marine Glycobiology recently won the European Research Council’s Consolidator Grant to research carbon storage in marine algal polysaccharides and their role in the marine carbon cycle.
- Work from Mukul Sharma’s lab at Dartmouth College is investigating the use of clay minerals to enhance the removal of carbon from the euphotic zone and sequester it in the deep ocean 10. The principal idea behind this research is the formation of clay-gel hybrids when clay comes into contact with dissolved organic matter produced by microalgae and bacteria. These hybrids may facilitate conversion of dissolved organic matter to particulate organic matter and rapid export out of the mixed layer. While clay additions are very underexplored in open ocean environments, they have long been recognized to interact with, and flocculate, in the presence of microalgae 1112.
-
- Long-lived bioproducts: wherein carbon sequestered in microalgae is incorporated into products such as bioplastics for long-term storage.
- Biochar: Microalgal biomass can be processed via pyrolysis, gasification, or hydrothermal carbonization and turned into biochar. Biochar is a carbon-rich product that can be used in soils for agriculture (helping to prevent leaching of pesticides and nutrients) or as absorbents in air/water treatments for pollutant removal 131415.
- Burial & land-based sequestration: Algal biomass that is sufficiently salty, dry, acidic, and rich in natural preservatives can be stored on land (buried) for long-term sequestration 16.
- Hazlebeck, David, and Rickman, William. Zobi® based processes for ultra-low energy algal harvesting and dewatering. United States: N. p., 2019.
- Muylaert, K., Bastiaens, L., Vandamme, D., & Gouveia, L. (2017). Harvesting of microalgae: Overview of process options and their strengths and drawbacks. Microalgae-Based Biofuels and Bioproducts, 113-132. https://doi.org/10.1016/B978-0-08-101023-5.00005-4
- Morales, Marjorie, et al. “Rotating Algal Biofilm versus Planktonic Cultivation: LCA Perspective.” Journal of Cleaner Production, vol. 257, 2020, p. 120547., https://doi.org/10.1016/j.jclepro.2020.120547.
- Morales, Marjorie, et al. “Rotating Algal Biofilm versus Planktonic Cultivation: LCA Perspective.” Journal of Cleaner Production, vol. 257, 2020, p. 120547., https://doi.org/10.1016/j.jclepro.2020.120547.
- Mantzorou A, Ververidis F. Microalgal biofilms: A further step over current microalgal cultivation techniques. Sci Total Environ. 2019 Feb 15;651(Pt 2):3187-3201. doi: 10.1016/j.scitotenv.2018.09.355. Epub 2018 Oct 1. PMID: 30463168.
- Li, Shengnan, et al. “How to Enhance Carbon Capture by Evolution of Microalgal Photosynthesis?” Separation and Purification Technology, vol. 291, June 2022, p. 120951, https://doi.org/10.1016/j.seppur.2022.120951.
- Siegel, D A, et al. 2021b. “Assessing the Sequestration Time Scales of Some Ocean-Based Carbon Dioxide Reduction Strategies.” Environmental Research Letters, vol. 16, no. 10, 2021b, p. 104003., https://doi.org/10.1088/1748-9326/ac0be0.
- Siegel, D A, et al. 2021b. “Assessing the Sequestration Time Scales of Some Ocean-Based Carbon Dioxide Reduction Strategies.” Environmental Research Letters, vol. 16, no. 10, 2021b, p. 104003., https://doi.org/10.1088/1748-9326/ac0be0.
- Nowicki, M., DeVries, T., & Siegel, D. A. (2022). Quantifying the carbon export and sequestration pathways of the ocean's biological carbon pump. Global Biogeochemical Cycles, 36, e2021GB007083. https://doi.org/10.1029/2021GB00708.
- Bates, E., et al. Role of Clay-Gel Hybrids in Enhancing Carbon Sequestration. Dec. 2019, pp. GC31I-1325, https://ui.adsabs.harvard.edu/abs/2019AGUFMGC31I1325B.
- Avnimelech, Yoram, Bill W. Troeger, and Lester W. Reed. "Mutual flocculation of algae and clay: evidence and implications." Science 216.4541 (1982): 63-65.
- Chen C, Pan G, Shi W, Xu F, Techtmann SM, Pfiffner SM and Hazen TC (2018) Clay Flocculation Effect on Microbial Community Composition in Water and Sediment. Front. Environ. Sci. 6:60. doi: 10.3389 fenvs.2018.00060
- Bi, Z., & He, B. B. (2022). Biochar from microalgae. 3rd Generation Biofuels, 613-637. https://doi.org/10.1016/B978-0-323-90971-6.00025-5
- Lee, Xin Jiat, et al. “State of Art Review on Conventional and Advanced Pyrolysis of Macroalgae and Microalgae for Biochar, Bio-Oil and Bio-Syngas Production.” Energy Conversion and Management, vol. 210, 2020, p. 112707., https://doi.org/10.1016/j.enconman.2020.112707.
- Wang, Liuwei, et al. “Role of Biochar toward Carbon Neutrality.” Carbon Research, vol. 2, no. 1, 2023, https://doi.org/10.1007/s44246-023-00035-7.
- OpenAir. (December 2022) .This Is CDR Ep. 57: Brilliant Planet with Dr. Raffael Jovine. [Video]. [YouTube]. https://www.youtube.com/watch?v=6B_LLBcdOhI&t=210s.
Technologies for Microalgae CDR
Nutrient Fertilization
CDR Potential: >1 Gt CO2/year 12, 0.1 – 1.0+ Gt CO2/year 3 Technology Readiness: Field studies still necessary to determine fate of carbon, net carbon sequestration, and sequestration durability 45 Nutrient fertilization refers to the addition of micronutrients (e.g., iron) and/or macronutrients (e.g., phosphorous, nitrogen, silica) to the ocean surface to increase photosynthesis by marine phytoplankton and, in turn, accelerate the uptake of carbon dioxide from seawater and enhance the transfer of organic carbon down the water column for sequestration. Note that there is a larger research focus on micronutrient fertilization (e.g., iron) because less nutrient is needed to sequester the same amount of carbon, making it more financially feasible and scalable. While increased photosynthetic uptake of CO2 via ocean nutrient fertilization has been well studied and documented for decades, the transfer of carbon from the atmosphere to the deep ocean resulting in sequestration is a challenge to measure, and to date has not been effectively demonstrated for a deliberately perturbed system 6. Long-term sequestration depends on a variety of factors including location, export depth, and the remineralization rates of the sinking particles, all of which need additional research. The ultimate CDR potential of large-scale microalgal cultivation and sequestration in the open ocean with nutrient fertilization is currently difficult to determine, in large part because many original scientific studies were not designed or well suited to measure carbon sequestration efficacy or durability 7. A broad review suggests that cultivating and sequestering microalgae could be scaled to 3.67 Gt CO2 equivalent (1 Gt of carbon) removed per year 8, however, in practice CDR may vary based on a variety of factors including:- The depth of remineralization for carbon, iron, and other macronutrients 9.
- Factors affecting carbon sequestration that remain uncertain 10:
- Net primary productivity in the vicinity of nutrient fertilization and downstream (to address issues associated with “nutrient robbing”).
- Efficiency of export of materials out of the euphotic zone, related to the attenuation of the particle flux
- Ratio of iron to carbon (Fe:C) in exported materials and how that ratio changes with depth
- Ecosystem / trophic level interactions
- The effectiveness of particular methods of delivering nutrients
- Pulse (one-time) versus continuous (repeated) experiments will likely change carbon capture efficiency
- Field-trials have yet to observe full growth cycles of microalgae including the bloom demise
- Artificial iron fertilization: The first observations of a relationship between iron and atmospheric CO2 came from geological records that revealed a correlation between CO2 in ice cores and iron rich dust 11. John Martin conducted pioneering work in the late 1980s to identify iron-limited regions of the ocean. The next decade (1993 – 2004) was spent executing studies in the Equatorial Pacific, Southern Ocean, Subarctic North Pacific, and Subarctic North Atlantic to explicitly examine the primary biological response of adding iron to surface waters. What resulted were thirteen studies in the open ocean accompanied by decades of analysis 12, rendering artificial iron fertilization the most studied and well-understood approach in this road map. The application of iron to the ocean surface to investigate the relationship between iron limitation and phytoplankton growth suggests potentially high global capacity for iron fertilization to result in gigaton scale carbon dioxide sequestration 131415 and has led scientists to look to iron as a strategy to enhance the ocean’s biological carbon pump 1617. Most of the open ocean experiments conducted to date were largely focused on the immediate biological response to added iron in the form of a microalgae bloom and not the geochemical response (in the form of carbon fluxes), thus there is limited knowledge on the efficacy of this technique for net carbon dioxide removal. However, there are a handful of groups around the globe currently investigating the fate of carbon from net primary production. In addition to the efficacy of such methods, biogeochemical and ecological impacts remain unknown. A 2022 whitepaper by ExOIS (Exploring Ocean Iron Solutions) lays out a number of questions that remain to be answered by science including: What controls the efficacy and durability of ocean iron fertilization as a CDR strategy? How can ocean iron fertilization efficacy be accurately and efficiently monitored and verified? What are the intended and unintended ecological consequences of ocean iron fertilization and how can they be mitigated?
-
- Engineered nanoparticles: Babakhani et al. (2021) propose the application of engineered nanoparticles in addition to iron fertilization in an attempt to enhance phytoplankton growth and possibly mitigate environmental risks posed by iron fertilization (e.g., nutrient robbing). Here, the goal is for the engineered nanoparticles to control the rate of nutrient release. Nanoparticles may be more expensive to deploy but may also be more effective at CDR. Focused field trials of engineered nanoparticles with artificial ocean fertilization are still needed to fully understand the impacts and efficacy of this technique.
-
- Triggering the formation of marine snow: The GEA275 Project (Croatia) is investigating if adding iron chelates to high-nitrate, low-chlorophyll regions of the ocean can induce the formation of marine snow (organic detritus) for carbon removal and storage in the deep sea. Unlike other forms of iron fertilization, this approach does not aim to stimulate phytoplankton growth but rather to accelerate the release of extracellular organic carbon by existing communities of phytoplankton and heterotrophic bacteria.
- Macronutrient fertilization: Compared to iron fertilization, macronutrient fertilization has received less attention from the scientific community (but note Harrison 2017), with one clear disadvantage of this method being that larger quantities of nutrient material are needed per ton of carbon removal due to the higher cellular ratios of nitrogen and phosphorus to carbon (as compared to iron to carbon). However, ideal locations for macronutrient fertilization (low-nutrient, low-chlorophyll) may be more accessible than hard-to-reach locations ideal for iron fertilization, such as the Southern Ocean. In general, macronutrient fertilization presents general concerns around acquiring sufficient quantities of nutrients (some of which are nonrenewable, like phosphate), ecosystem impacts, and the fate of carbon once sequestered in phytoplankton. More work is needed to better understand macronutrient fertilization’s potential as a scalable CDR technique 18.
Artificial Upwelling
CDR Potential: <0.1 Gt CO2/year. There is low confidence that artificial upwelling will be effective, durable, or scalable for CDR 19 Technology Readiness: The technology necessary to conduct field trials is not ready for deployment 20. Artificial upwelling, the pumping of nutrient rich water to the surface for increased photosynthetic activity, has been proposed as a mechanism by which to remove atmospheric carbon dioxide. In general, the overall CDR potential of artificial upwelling is thought to be low because of the coincident upwelling of high CO2 water alongside nutrients and the potential for off gassing. Off gassing of upwelled high CO2 deep water from the ocean to the atmosphere is anticipated to offset some or all the carbon sequestration supported by the introduction of new nutrients into the surface ocean. The NASEM Report (2022) on ocean-based carbon dioxide removal estimated that artificial upwelling / artificial downwelling projects could remove and sequester between 0.1 and 1.0 gigatons of atmospheric carbon dioxide per year, noting however, that estimates are limited to modeling studies and there exists no proof of concept that artificial upwelling could sequester carbon below the pycnocline. A 2022 modelling study of artificial upwelling systems with current technology estimated global potential for carbon dioxide removal using microalgae was less than 50 megatons (0.05 gigatons) of carbon dioxide annually 21. Pilot-scale field trials that examine carbon flux are needed to assess potential efficacy of artificial upwelling as a CDR approach. Other barriers to this technology include high costs of energy, construction, and maintenance, and a 2016 review paper by Pan et al. found that while devices have been successfully deployed in the field for months at a time, they pose a significant disturbance to ecosystems which limits scaling potential as well as introducing many unknown ecosystem risks.Floating Photobioreactors
CDR Potential: unknown Technology Readiness: Many designs and configurations have been proposed, but more field trials are needed to assess carbon capture efficiency and scalability, as well as address the issue of carbon supply. Floating photobioreactors are systems for cultivating microalgae that are either stationary or towed behind ships in the open ocean. These systems, while still in early stages of development, show great potential of efficient microalgal production with low manufacturing and operating costs. This is primarily attributed to lower fabrication, operating, and maintenance costs than conventional cultivation systems, free wave energy to provide mixing, and nutrients, space, and temperature controlled by the surrounding water 22. Several designs have been proposed which effectively incorporate photobioreactors as floating platforms towed behind ships. These photobioreactors are powered by wave energy and incorporate nutrients from the ocean water as inputs into the microalgal cultivation 232425. Currently, the biggest challenge to commercialization for floating photobioreactors technology is carbon supply which is typically supplied by aerating CO2-enriched air and does not represent a carbon negative pathway (but rather a carbon neutral pathway). One example of past work done in this field is the NASA Offshore Membrane Enclosures for Growing Algae (OMEGA) Project. This project deployed plastic enclosures out in the water with photobioreactors incorporating semi permeable membranes that allow for osmosis from saltwater to freshwater and allowing the water to provide cooling and mixing from wave action, avoiding land costs and incorporating wastewater rich in nutrients and CO2. Such technology could be adapted in future iterations to be towed behind ships.- Combining cultivation with wastewater treatment: Wastewater is a nutrient-rich medium that has been explored as a nutrient source for microalgae cultivation 26. Wastewater is rich in nitrogen, phosphorous, heavy metals, etc., which are often costly and energy-intensive to remove through traditional methods 27. Many species of microalgae can remove these compounds by accumulating and/or using them in their cells. One challenge in this approach is providing microalgae with enough CO2 for optimal growth. There is potential for wastewater treatment to be paired with onshore cultivation systems such as raceway ponds or with offshore systems such as photobioreactors, however more research is needed to assess the feasibility of such set-ups. Most studies into coupling wastewater treatment with microalgae have looked at end products such as biofuels and not the permanent removal of carbon dioxide. As such, there is limited data on the actual carbon dioxide removal potential of this approach. While closed microalgae cultivation is widely considered to be an economically non-viable way to make fuels 2829, it may be viable for CDR purposes.
-
- Current Projects: Algae Systems (US) grows microalgae in bags of disinfected wastewater and CO2. The bags are deployed offshore in Mobile Bay, Alabama, and the combination of wave energy and sun create the necessary conditions for algae to grow. Currently, the algae are harvested and used for diesel and jet fuel, while the water is cleaned for re-use. The plant is currently at demo-scale and treats 40,000 gallons of wastewater per acre per day.
Cultivation Ponds
CDR Potential: Largely unknown, Brilliant Planet projects 5 Gt CO2/year 30 Technology Readiness: Techniques and methods are well studied, and many are well established (though very few have been intended for CDR). Raceway ponds are largely ready to be scaled. Onshore ponds in which microalgae is cultivated through controlled inputs of water and nutrients are the most established method of cultivating microalgae. More frequently, cultivation ponds are used to grow microalgae for end uses other than carbon removal, such as for high value bioproducts for use in cosmetics, food, and feed. Recently, there has been growing interest in using seawater to grow microalgae on land and sequester the carbon as a means of reducing atmospheric CO2. While the technology for growing microalgae on land is relatively well developed, questions remain around scalability due to many factors like competition with other land-uses/land-users and the cost of water and energy. Although closed microalgae cultivation is considered an economically non-viable way to make biofuel 31, these same barriers may not apply to CDR where the “final product” has an entirely different value.- Current Projects: Brilliant Planet (London) began growing microalgae in 2013 on the shores of St Helena, South Africa and now have a thirty-thousand square meter production facility in the coastal desert of Morocco which has been operating for over five years. Their systems use seawater to cultivate microalgae in large ponds and are monitored with high-frequency satellites. Permanent carbon sequestration is achieved by burying resulting biomass in ultra-dry desert landfills and sealing it with a geomembrane liner 32
Microalgae-based Bioenergy for Carbon Capture and Storage (MBECCS / ABECCS)
CDR Potential: unknown Technology Readiness: Early stages of development Microalgae production can be paired with ‘bioenergy with carbon capture and storage’ (BECCS) wherein microalgae is used as the source of bioenergy and its associated carbon is sequestered for long-term storage. Traditionally, BECCS takes terrestrial plants that absorb atmospheric CO2 via photosynthesis and then extracts the energy embedded in the biomass while the resulting CO2 is captured and stored. Microalgae are an intriguing substitution for terrestrial plants due to their high photosynthetic efficiencies. Even et al. (2022) models the firing of microalgae grown with only atmospheric CO2 and shows that MBECCS uses four times less cultivation area than BECCS. If this method can become cost-effective, it would increase the scalability of bioenergy with carbon capture and storage by decoupling it from land use. Another way of pairing microalgae with BECCS is by injecting the CO2 captured through traditional BECCS into microalgae ponds to increase productivity. Beal et al. (2018a) modeled a system that paired a 121-hectare algae facility with a 2,680-hectare eucalyptus forest for algae-based bioenergy with carbon capture and storage (ABECCS), sequestering 29,600 t of CO2 per year. Theoretically, the carbon captured by the microalgae could be durably sequestered in the ocean, however, it should be noted that in these models, microalgae were used for high value bioproducts and may not count as CDR.- Current Projects: The Johnson Lab at the Duke Nicholas School of the Environment was awarded a grant for a pilot demonstration of carbon capture/storage technology based on ABECCS. The project uses a combined system that generates CO2 from forest products (bark/sawdust) and then uses that CO2 to grow algae for carbon sequestration in valuable bioproducts. Preliminary analysis of this process shows it to be carbon negative and economically viable.
- NASEM (National Academies of Sciences, Engineering, and Medicine.) A Research Strategy for Ocean-Based Carbon Dioxide Removal and Sequestration. The National Academies Press, https://doi.org/10.17226/26278. Accessed 18 Aug. 2022.
- GESAMP (2019). “High level review of a wide range of proposed marine geoengineering techniques”. (Boyd, P.W. and Vivian, C.M.G., eds.). (IMO/FAO/UNESCO-IOC/UNIDO/WMO/IAEA/UN/UN Environment/ UNDP/ISA Joint Group of Experts on the Scientific Aspects of Marine Environmental Protection). Rep. Stud. GESAMP No. 98, 144 p.
- NOAA, 2022. A White Paper documenting a Potential NOAA CDR Science Strategy as an element of NOAA’s Climate Mitigation Portfolio. https://sciencecouncil.noaa.gov/Draft-CDR-Strategy.
- NASEM (National Academies of Sciences, Engineering, and Medicine.) A Research Strategy for Ocean-Based Carbon Dioxide Removal and Sequestration. The National Academies Press, https://doi.org/10.17226/26278. Accessed 18 Aug. 2022.
- ExOIS, 2022. Ocean Iron Fertilization: Assessing its potential as a climate solution. https://cafethorium.whoi.edu/ocean-iron-fertilization-assessing-its-potential-as-a-climate-solution/
- NASEM (National Academies of Sciences, Engineering, and Medicine.) A Research Strategy for Ocean-Based Carbon Dioxide Removal and Sequestration. The National Academies Press, https://doi.org/10.17226/26278. Accessed 18 Aug. 2022.
- Buesseler, Ken O., et al. “Ocean Iron Fertilization--Moving Forward in a Sea of Uncertainty.” Science, vol. 319, no. 5860, Jan. 2008, pp. 162–162, https://doi.org/10.1126/science.1154305.
- GESAMP (2019). “High level review of a wide range of proposed marine geoengineering techniques”. (Boyd, P.W. and Vivian, C.M.G., eds.). (IMO/FAO/UNESCO-IOC/UNIDO/WMO/IAEA/UN/UN Environment/ UNDP/ISA Joint Group of Experts on the Scientific Aspects of Marine Environmental Protection). Rep. Stud. GESAMP No. 98, 144 p.
- NASEM (National Academies of Sciences, Engineering, and Medicine.) A Research Strategy for Ocean-Based Carbon Dioxide Removal and Sequestration. The National Academies Press, https://doi.org/10.17226/26278. Accessed 18 Aug. 2022.
- NASEM (National Academies of Sciences, Engineering, and Medicine.) A Research Strategy for Ocean-Based Carbon Dioxide Removal and Sequestration. The National Academies Press, https://doi.org/10.17226/26278. Accessed 18 Aug. 2022.
- Martin, J. H., R. M. Gordon, and S. E. Fitzwater. 1990. Iron in Antarctic waters. Nature 345(6271):156-158. doi:10.1038/345156a0.
- Yoon, Joo-Eun, et al. "Reviews and syntheses: Ocean iron fertilization experiments–past, present, and future looking to a future Korean Iron Fertilization Experiment in the Southern Ocean (KIFES) project." Biogeosciences 15.19 (2018): 5847-5889.
- Martin, J. H., and S. E. Fitzwater. 1988. Iron deficiency limits phytoplankton growth in the north-east Pacific subarctic. Nature 331(6154):341-343. doi:10.1038/331341a0.
- Morel, F. M., and N. M. Price. 2003. The biogeochemical cycles of trace metals in the oceans. Science 300(5621):944-947. doi:10.1126/science.1083545.
- ExOIS, 2022. Ocean Iron Fertilization: Assessing its potential as a climate solution. https://cafethorium.whoi.edu/ocean-iron-fertilization-assessing-its-potential-as-a-climate-solution/
- Martin, J. H., R. M. Gordon, and S. E. Fitzwater. 1990. Iron in Antarctic waters. Nature 345(6271):156-158. doi:10.1038/345156a0.
- Martin, J. H., and S. E. Fitzwater. 1988. Iron deficiency limits phytoplankton growth in the north-east Pacific subarctic. Nature 331(6154):341-343. doi:10.1038/331341a0.
- NASEM (National Academies of Sciences, Engineering, and Medicine.) A Research Strategy for Ocean-Based Carbon Dioxide Removal and Sequestration. The National Academies Press, https://doi.org/10.17226/26278. Accessed 18 Aug. 2022.
- NASEM (National Academies of Sciences, Engineering, and Medicine.) A Research Strategy for Ocean-Based Carbon Dioxide Removal and Sequestration. The National Academies Press, https://doi.org/10.17226/26278. Accessed 18 Aug. 2022.
- NASEM (National Academies of Sciences, Engineering, and Medicine.) A Research Strategy for Ocean-Based Carbon Dioxide Removal and Sequestration. The National Academies Press, https://doi.org/10.17226/26278. Accessed 18 Aug. 2022.
- Koweek, D. A. (2021). Expected Limits on the Potential for Carbon Dioxide Removal From Artificial Upwelling. Frontiers in Marine Science. https://doi.org/10.3389/fmars.2022.841894
- Zhu, Chenba, et al. “Efficient CO2 Capture from the Air for High Microalgal Biomass Production by a Bicarbonate Pool.” Journal of CO2 Utilization, vol. 37, 2020, pp. 320–327., https://doi.org/10.1016/j.jcou.2019.12.023.
- Zhai, Xiaoqian, et al. “Seawater Supplemented with Bicarbonate for Efficient Marine Microalgae Production in Floating Photobioreactor on Ocean: A Case Study of Chlorella Sp.” Science of The Total Environment, vol. 738, Oct. 2020, p. 139439, https://doi.org/10.1016/j.scitotenv.2020.139439.
- Kim, Z.-Hun, et al. “Development of a Floating Photobioreactor with Internal Partitions for Efficient Utilization of Ocean Wave into Improved Mass Transfer and Algal Culture Mixing.” Bioprocess and Biosystems Engineering, vol. 39, no. 5, May 2016, pp. 713–23, https://doi.org/10.1007/s00449-016-1552-6.
- Zhu, Chenba, et al. “Bicarbonate-Based Carbon Capture and Algal Production System on Ocean with Floating Inflatable-Membrane Photobioreactor.” Journal of Applied Phycology, vol. 30, no. 2, Apr. 2018, pp. 875–85, https://doi.org/10.1007/s10811-017-1285-1.
- Wang, S., Mukhambet, Y., Esakkimuthu, S., & Abomohra, A. E. F. (2022). Integrated microalgal biorefinery – Routes, energy, economic and environmental perspectives. Journal of Cleaner Production, 348, 131245. https://doi.org/10.1016/j.jclepro.2022.131245
- Molazadeh M, Ahmadzadeh H, Pourianfar HR, Lyon S and Rampelotto PH (2019) The Use of Microalgae for Coupling Wastewater Treatment With CO2 Biofixation. Front. Bioeng. Biotechnol. 7:42. doi: 10.3389/fbioe.2019.00042
- Kumar, D., & Singh, B. (2019). Algal biorefinery: An integrated approach for sustainable biodiesel production. Biomass and Bioenergy, 131, 105398. https://doi.org/10.1016/j.biombioe.2019.105398
- Wang, S., Mukhambet, Y., Esakkimuthu, S., & Abomohra, A. E. F. (2022). Integrated microalgal biorefinery – Routes, energy, economic and environmental perspectives. Journal of Cleaner Production, 348, 131245. https://doi.org/10.1016/j.jclepro.2022.131245
- OpenAir. (December 2022) .This Is CDR Ep. 57: Brilliant Planet with Dr. Raffael Jovine. [Video]. [YouTube]. https://www.youtube.com/watch?v=6B_LLBcdOhI&t=210s.
- Lane, T. W. (2022). Barriers to microalgal mass cultivation. Current Opinion in Biotechnology, 73, 323-328. https://doi.org/10.1016/j.copbio.2021.09.013
- OpenAir. (December 2022) .This Is CDR Ep. 57: Brilliant Planet with Dr. Raffael Jovine. [Video]. [YouTube]. https://www.youtube.com/watch?v=6B_LLBcdOhI&t=210s.
Environmental Impacts
General Risks of Open Systems
Ocean fertilization and artificial upwelling are, in part, characterized by intentionally perturbing the natural ecosystem to encourage an increase of photosynthesis and the production of organic matter. Approaches that take place in the open ocean will very likely impact the surrounding ecosystems and ecology, including biological, chemical, and physical changes. As such, these disturbances will very likely change species composition and biodiversity. Other downstream impacts include potential for ocean acidification, hypoxia, the production of greenhouse gases, and mid-water oxygen depletion. Ocean fertilization and artificial upwelling share many of the same potential environmental impacts (risks and co-benefits) with a key difference being that artificial upwelling also affects the ocean’s density field and sea surface temperature, and ecological shifts would likely result from the influx of cold, nutrient-rich waters to the ocean surface 1. While these potential impacts have either been observed from models or during small-scale field experiments, more controlled field trials are necessary for a better characterization of environmental impacts from artificial upwelling.Harmful Algal Blooms
One of the most prominent concerns with artificial iron fertilization is the possibility that it will increase the abundance of Pseudonitzschia, a genus of diatom that is known to produce the neurotoxin domoic acid, leading to a harmful algal bloom (HAB). However, there is not data that suggest significantly higher levels of domoic acid after an artificial iron fertilization event 234. While it’s possible that HABs may occur on land in pond cultivation systems, risks from contained systems would likely be far easier to manage and control.Land-Use
An obvious difference between onshore and offshore approaches is land use. Offshore methods of cultivating microalgal growth require no land, although will likely face barriers in the form of permitting and governance. Onshore cultivation methods such as ponds will require vast swaths of land to scale meaningfully. This presents a number of environmental impacts including land use change and the energy required to clear areas and build and operate facilities. All these impacts will need to be fully considered when assessing true net carbon removal as well as tolerance for environmental change. Onshore cultivation may also pose a risk to coastal habitats from spills, leakage, and ruptures.Summary Table of Potential Impacts
Below is table with a summary of some of the possible risks and co-benefits associated with multiple approaches as well as an estimate of their likelihood of occurring and the severity of resulting consequences. It is important to note that environmental risks likely vary greatly across scales. For example, a pilot-scale experiment likely brings about substantially lower risks and less severe consequences than a commercial operation.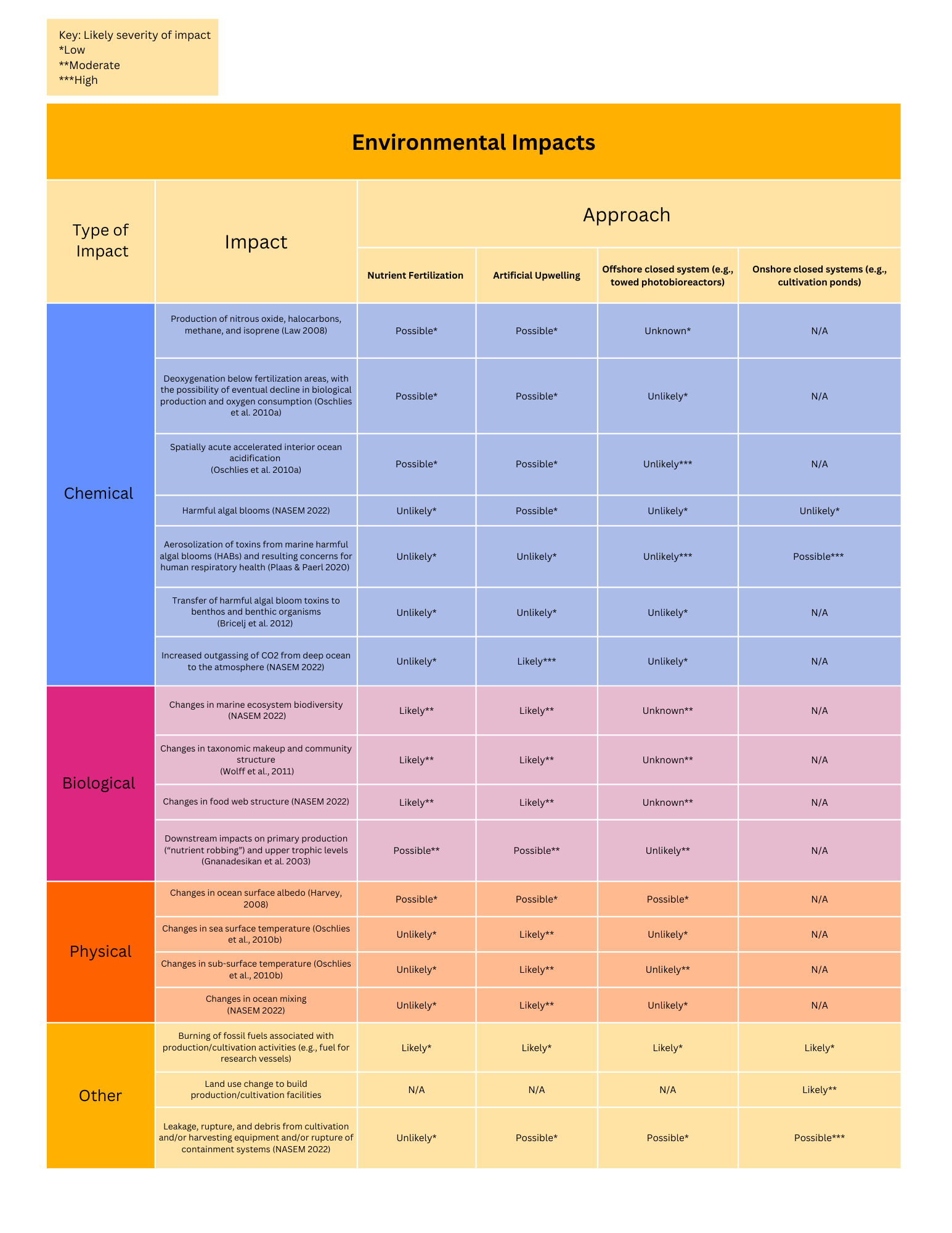
- NASEM (National Academies of Sciences, Engineering, and Medicine.) A Research Strategy for Ocean-Based Carbon Dioxide Removal and Sequestration. The National Academies Press, https://doi.org/10.17226/26278. Accessed 18 Aug. 2022.
- Marchetti, A., M. S. Parker, L. P. Moccia, E. O. Lin, A. L. Arrieta, F. Ribalet, M. E. P. Murphy, M. T. Maldonado, and E. V. Armbrust. 2009. Ferritin is used for iron storage in bloom-forming marine pennate diatoms. Nature 457(7228):467- 470. doi:10.1038/nature07539.
- Trick, C. G., B. D. Bill, W. P. Cochlan, M. L. Wells, V. L. Trainer, and L. D. Pickell. 2010. Iron enrichment stimulates toxic diatom production in high-nitrate, low-chlorophyll areas. Proceedings of the National Academy of Sciences of the United States of America 107(13):5887-5892. doi:10.1073/pnas.0910579107.
- Silver, M. W., S. Bargu, S. L. Coale, C. R. Benitez-Nelson, A. C. Garcia, K. J. Roberts, E. Sekula-Wood, K. W. Bruland, and K. H. Coale. 2010. Toxic diatoms and domoic acid in natural and iron enriched waters of the oceanic Pacific. Proceedings of the National Academy of Sciences of the United States of America 107(48):20762-7. doi:10.1073/ pnas.1006968107.
Social Impacts
Social Risks
- Lack of public support & social license: There are currently low levels of public support and/or acceptance of CDR broadly in the United States and beyond, particularly with activities viewed as “geoengineering” or “climate engineering” 34. Other factors that may affect public support include perceptions of scale and perceptions of capture versus storage. These are areas that require more focused study to better understand. Early experiments are opportunities to adopt best practices and avoid mistakes when building public support and stakeholder engagement.
- Risk to culturally significant landscapes: CDR interventions that alter marine landscapes may lead to disruptions (e.g., native species populations) to cultural, spiritual, or cosmological landscapes and other non-tangible uses or relationships to the ocean and its inhabitants. The introduction of any new technology that interacts with natural ecosystems is likely to always be contested due to the diversity of ways in which people view the relationship between humans and the non-human world 56.
- Human health risks: Exposure to aerosolization of toxins from harmful algal blooms (HABs) have been known to cause respiratory symptoms in humans 7. Although HABs have not been observed during artificial iron fertilization experiments, their occurrence is not impossible across many microalgae-based CDR approaches, including shore-based cultivation operations.
- Irreversible consequences: Negative impacts (environmental or social) of an approach that are irreversible may impact future generations, raising concerns regarding intergenerational justice 8. This concern highlights the need for appropriate procedures so that any field trials that are approved and deployed do not have irreversible consequences.
- Decreased equity and social welfare due to financing: The creation of financing systems like carbon markets for forest carbon sequestration caused tensions around land rights and had implications on human rights and equity for Indigenous and forest-dependent communities 9. These issues will likely vary greatly for closed systems on land, coastal closed systems, and open ocean/deep ocean commons.
- Location considerations: Just as there are different governance implications for activities occurring in exclusive economic zones versus the high seas, these geographical differences may also carry importance for social impacts. Environmental or ecological impacts from activities taking place in near-shore waters may have more direct social impacts than activities taking place in the open ocean.
- Food security: Microalgae-based interventions that risk disturbing food supply chains (e.g., fisheries) may threaten food security. This is especially relevant for communities that rely on the ocean for sustenance.
- Impacts on livelihoods from tourism and fisheries: Activities that use or interfere with near-shore environments and ecology may impact industries like tourism and fisheries, thus impacting livelihoods. This is particularly important in areas that depend heavily on these industries economically and/or are socioeconomically vulnerable in general (e.g., the Global South).
Social Co-Benefits
- Economic incentives: The various potential utilization pathways of microalgae could create avenues for wealth generation and emissions-free economic growth – namely economic incentives within existing carbon markets, preventing costly environmental disasters resulting from uncontrolled climate change, and converting a damaging waste product (CO2) into a value-added product. 10
- Job creation: Economic value-addition is likely to also create jobs in new and existing industries in aquaculture and beyond 11.
- Ownership and benefits accruing from uses of genetic resources: Many microalgae-based innovations bring with them a suite of potential biotech and genomic-based approaches. It will be important to understand when and how local benefits rights holders (e.g., coastal communities, Indigenous communities) can benefit from the use of marine-based genetic resources. This is an inherently complex subject that questions ownership of marine resources and is rich with justice and equity implications.
- Gannon, Kate and Hulme, Mike (2018) Geoengineering at the 'edge of the world': exploring perceptions of ocean fertilization through the Haida Salmon Restoration Corporation. Geo: Geography and Environment, 5 (1). e00054. ISSN 2054-4049 DOI: 10.1002/geo2.54
- Loomis R, Cooley SR, Collins JR, Engler S and Suatoni L (2022) A Code of Conduct Is Imperative for Ocean Carbon Dioxide Removal Research. Front. Mar. Sci. 9:872800. doi: 10.3389/fmars.2022.872800
- Carlisle, Daniel P., et al. “The Public Remain Uninformed and Wary of Climate Engineering.” Climatic Change, vol. 160, no. 2, 2020, pp. 303–322., https://doi.org/10.1007/s10584-020-02706-5.
- Cox, Emily, et al. “Public Perceptions of Carbon Dioxide Removal in the United States and the United Kingdom.” Nature Climate Change, vol. 10, no. 8, 2020, pp. 744–749., https://doi.org/10.1038/s41558-020-0823-z.
- Gannon, Kate and Hulme, Mike (2018) Geoengineering at the 'edge of the world': exploring perceptions of ocean fertilization through the Haida Salmon Restoration Corporation. Geo: Geography and Environment, 5 (1). e00054. ISSN 2054-4049 DOI: 10.1002/geo2.54, https://rgs-ibg.onlinelibrary.wiley.com/doi/full/10.1002/geo2.54
- Abate, Randall S. “Ocean Iron Fertilization and Indigenous Peoples’ Right to Food: Leveraging International and Domestic Law Protections to Enhance Access to Salmon in the Pacific Northwest.” UCLA Journal of International Law and Foreign Affairs, vol. 20, 2016, p. 45, https://heinonline.org/HOL/Page?handle=hein.journals/jilfa20&id=49&div=&collection=.
- Stewart, Ian, et al. “Recreational and Occupational Field Exposure to Freshwater Cyanobacteria – a Review of Anecdotal and Case Reports, Epidemiological Studies and the Challenges for Epidemiologic Assessment.” Environmental Health, vol. 5, no. 1, 2006, https://doi.org/10.1186/1476-069x-5-6.
- Cooley, Sarah R., et al. “Sociotechnical Considerations about Ocean Carbon Dioxide Removal.” Annual Review of Marine Science, vol. 15, no. 1, 2022, https://doi.org/10.1146/annurev-marine-032122-113850.
- Cooley, Sarah R., et al. “Sociotechnical Considerations about Ocean Carbon Dioxide Removal.” Annual Review of Marine Science, vol. 15, no. 1, 2022, https://doi.org/10.1146/annurev-marine-032122-113850.
- Daneshvar, Ehsan, et al. “Biologically-Mediated Carbon Capture and Utilization by Microalgae towards Sustainable CO2 Biofixation and Biomass Valorization – A Review.” Chemical Engineering Journal, vol. 427, Jan. 2022, p. 130884, https://doi.org/10.1016/j.cej.2021.130884.
- Mayo-Ramsay, Julia. “Environmental, Legal and Social Implications of Ocean Urea Fertilization: Sulu Sea Example.” Marine Policy, vol. 34, no. 5, 2010, pp. 831–835., https://doi.org/10.1016/j.marpol.2010.01.004.
Policy and Governance
Existing Governance Structures that May be Applicable to Microalgae for CDR
- United Nations Convention on the Law of the Sea (UNCLOS): Adopted/ratified by 167 countries and the European Union, UNCLOS defines countries’ rights and responsibilities in terms of use and management of offshore areas. Provisions that may be relevant to microalgae cultivation and/or sequestration in the open ocean include:
- Part XIII of UNCLOS which establishes rules for “marine scientific research”
- Part XII of UNCLOS which requires countries to “protect and preserve rare or fragile ecosystems as well as the habitat of depleted, threatened or endangered species and other forms of marine life” and includes a number of specific provisions on marine pollution from vessels, land-based sources, activities in the area, and dumping, among others. Without clear distinctions on what is or is not considered “pollution”, these provisions may be relevant for microalgae-based CDR interventions.
- Proelss (2022) investigates the United Nations Convention on the Law of the Sea and finds that although it covers ocean fertilization research stages, it does not apply to ocean fertilization for CDR, leaving the legality of ocean fertilization unclear under international law.
- Convention on Biological Diversity (CBD): Adopted in 1992, the CBD aims to protect biological diversity. 195 countries and the European Union have ratified it; the United States has signed but not ratified it. In 2008 the Conference of the Parties of the Convention on Biological Diversity recommended that ocean fertilization activities not take place until there exists a sufficient scientific basis to justify it. The term “ocean fertilization” was not defined. In 2010 a decision was made to regulate “geoengineering activities” such that no geoengineering activities take place that may impact biodiversity until there is a scientific basis to justify such actions. An exception is made for small-scale scientific experiments in controlled settings (the definition of “controlled settings” may prevent research in the open ocean) after thorough assessment and justification, though key terms in the exceptions for scientific experimentation are not defined, resulting in significant uncertainty as to when it will apply.
- London Protocol / London Convention: Adopted in 1972, this convention aimed to control all sources of pollution on the ocean, with particular focus on the dumping of waste or other materials in the ocean. There is a lack of clarity and general disagreement concerning what the future of policy and governance application of ocean iron fertilization may look like, particularly with the precedent of the London Protocol, which has banned nonscientific application outright1. The London Protocol has not yet ruled on closed offshore systems and represents an opportunity to bring attention back to issues of ocean utilization for carbon dioxide removal.
- Amendment on Ocean CDR: This amendment, not yet entered into force, states: “Contracting Parties shall not allow the placement of matter into the sea from vessels, aircraft, platforms or other man-made structures at sea for marine geoengineering activities listed in annex 4 (amendment that regulates the placement of matter into the ocean for marine geoengineering), unless the listing provides that the activity or the subcategory of an activity may be authorized under a permit.”
- Statement on Marine Geoengineering: In October of 2022, parties to the treaties which regulate marine dumping adopted a statement calling out the need for evaluation of four marine geoengineering techniques that have potential to mitigate the effects of climate change but could also threaten marine ecosystems. The four techniques given priority are: ocean alkalinity enhancement, macroalgae cultivation and sequestration (including artificial upwelling), marine cloud brightening, and microbubbles/reflective particles/materials. The London Protocol and London Convention Parties are conducting a technical and legal analysis to evaluate next steps and future regulation. (See the International Maritime Organization’s briefing)
- GESAMP (Group of Experts on the Scientific Aspects of Marine Environmental Protection) Working Group 41: Ocean Interventions for Climate Change (formerly Marine Geoengineering): In early 2022, GESAMP’s Working Group 41 formally advised the London Protocol Parties to consider marine geoengineering techniques to list in the new annex 4 of the protocol. These geoengineering techniques are those that may assist in mitigating or reversing the impacts of climate change. Included in this list are approaches that utilize microalgae to sequester carbon for long-term storage. (Timeline of Ocean Fertilization under the London Protocol)
Other Considerations
- Creation of new frameworks: In many cases it may be most efficient and effective to create new frameworks that put controls on research to ensure it occurs in a safe and responsible way that aren’t unnecessarily restrictive or hindering.
- Modification of existing policy: Examine, and consider reforming, existing policies that have the potential to inhibit the research and development of this technology, e.g., the London Protocol.
- Scale considerations: Pilot-scale experiments will likely have different associated risks than full-scale implementation. This may translate into policy and governance frameworks that consider the scale of manipulation or experimentation as a critical variable.
- Government subsidies: Subsidies from the government could provide incentive to researchers as well as the funds necessary to accelerate research.
- Bias amongst approaches: How will the ability to monitor and verify impacts and efficacy of a particular approach influence support from governance and policy? An approach-neutral stance (with financing, policy etc.) will help ensure that all approaches are given an opportunity to reach their full potential.
- Resource competition for onshore cultivation: There is potential for competition for nutrients with traditional agriculture and other societal priorities. Although microalgae can be grown on marginal lands unsuitable for agriculture, land competition with human settlement, industrial processes, and other infrastructure may pose a challenge if microalgae production is scaled up significantly (Scott-Buechler, in preparation).
- Using existing frameworks for onshore cultivation: Onshore cultivation of microalgae may look similar to established aquaculture or desalination plant policies/laws and thus have a more straightforward path for research and development.
- Rohr, Tyler. “Southern Ocean Iron Fertilization: An Argument Against Commercialization but for Continued Research Amidst Lingering Uncertainty.” Journal of Science Policy & Governance, Nov. 2019, https://doi.org/10.38126/JSPG150114.
Development Gaps and Needs
Overarching Knowledge Gaps
- Measurement, reporting, and verification (MRV) procedures will need to be developed following advances in research and development. Advances in research and development will inform what parameters need to be monitored and verified, and how to do so. MRV protocols should include measurements of key ecological parameters as well as carbon fluxes to ensure that microalgae CDR activities do not exceed accepted limits of environmental impact.
- Life cycle analyses will need to be conducted for any approach that uses microalgae for CDR to determine the net carbon removal potential after accounting for process and embedded emissions.
- Social co-benefits are not well understood or identified. While research into social impacts is growing, there remain large uncertainties around the range of potential impacts that may result from large-scale environmental interventions. Integrating social risks and co-benefits into CDR research agendas may help this area of knowledge grow alongside the technical research and development.
- Environmental co-benefits have not been well studied or articulated across microalgae-based CDR methods 1. Identifying and understanding the potential array of co-benefits may help offset other risks, both environmental and social, and aid in decisions around which methods are to be pursued further.
- Location and siting: Siting analyses are needed to identify optimal locations for microalgae CDR and address potential synergies and/or conflicts with other marine resource users (e.g., fisheries, renewable energy, etc.) (Map optimal locations for microalgae-based approaches and engage with interested and affected constituents)
- NASEM (National Academies of Sciences, Engineering, and Medicine.) A Research Strategy for Ocean-Based Carbon Dioxide Removal and Sequestration. The National Academies Press, https://doi.org/10.17226/26278. Accessed 18 Aug. 2022.
Natural and Physical Sciences
Open Ocean Systems
- Export and fate of carbon from net primary production in the upper ocean to understand sequestration efficacy and durability: A fundamental understanding of the export and fate of net primary production from the upper ocean is critical to any development, testing, or deployment of approaches that hope to accelerate this process. Currently a number of field campaigns are working on answering these questions, including EXPORTS (USA), COMICS (UK), and ONCE (China). In addition to these large campaigns focused on fundamental understanding of the biological carbon pump, these questions must be answered in the context of purposeful nutrient additions (Accelerate the design, permitting, and execution of the next generation of controlled field trials to answer questions specifically pertaining to carbon sequestration)
- Natural iron cycle / anthropogenic iron input: There is a lack of knowledge about the current state of iron cycling in the ocean system, particularly how much anthropogenic iron is already input into the system and what the spatially specific distribution of impacts are likely to be 1. Research must prioritize understanding inputs and refining existing iron-cycle models. This will be critical work for ocean iron chemistry models to measure future changes in anthropogenic iron loading. Future research must also seek to better understand natural and pre-existing anthropogenic analogs that may be analyzed as simulations of future ocean iron fertilization23. Analogs may include volcanic ash and wildfire ash deposits into ocean systems.
Closed Systems
- Harvesting efficiency: In the cultivation stage, harvesting microalgae from closed systems contributes to 20–30% of the total cost of microalgal biomass production, so opportunities to increase efficiency present the greatest opportunity for cost reductions through innovation 4. (Optimize and/or efficiently automate all approaches for scalability and economic feasibility)
- Strain optimization: The algal industry is still relatively small, and primarily optimizes for lipid and/or protein content in microalgae strains. More research is thus needed to identify ideal species, strains, and specific growth conditions to maximize microalgal CO2 uptake; and to estimate associated costs. (Optimize and/or efficiently automate all approaches for scalability and economic feasibility)
- Fertilizer efficiency: High fertilizer needs are likely to be the most substantial constraint on closed onshore cultivation systems5. Research is needed into options to maximize fertilizer recycling and other strategies to lessen fertilizer needs. (Optimize and/or efficiently automate all approaches for scalability and economic feasibility)
- Overall CDR potential: There exists virtually no information on overall CDR potential of closed offshore systems (e.g., towed photobioreactors). (Accelerate the design, permitting, and execution of the next generation of controlled field trials to answer questions specifically pertaining to carbon sequestration)
- Liu, Mingxu, et al. “The Underappreciated Role of Anthropogenic Sources in Atmospheric Soluble Iron Flux to the Southern Ocean.” Npj Climate and Atmospheric Science, vol. 5, no. 1, Apr. 2022, pp. 1–9, https://doi.org/10.1038/s41612-022-00250-w.
- Bach, Lennart T., and Philip W. Boyd. “Seeking Natural Analogs to Fast-Forward the Assessment of Marine CO 2 Removal.” Proceedings of the National Academy of Sciences, vol. 118, no. 40, Oct. 2021, p. e2106147118, https://doi.org/10.1073/pnas.2106147118.
- Weis, Jakob, et al. “Southern Ocean Phytoplankton Stimulated by Wildfire Emissions and Sustained by Iron Recycling.” Geophysical Research Letters, vol. 49, no. 11, June 2022, https://doi.org/10.1029/2021GL097538.
- Singh, U. B., & Ahluwalia, A. S. “Microalgae: a promising tool for carbon sequestration. Mitigation and Adaptation Strategies for Global Change, vol. 18, no. 1, 2013, pp. 73-95.
- Huntley, M.E., et al. (2015). Demonstrated large-scale production of marine microalgae for fuels and feed. Algal Research, 10, 249-265. https://doi.org/10.1016/j.algal.2015.04.016
Market Dynamics
- Mapping interested and affected constituencies: It will be important to identify the diverse stakeholders for microalgae CDR, including producers, potential buyers for carbon removal, potential buyers for other useful co-products, and communities where projects may be sited. Understanding perceptions, needs, concerns, and opportunities across multiple stakeholder groups is necessary to scale microalgae-based CDR.
- Industry transitions: Microalgae is already cultivated around the world today but is done so for non-CDR purposes—primarily feeds and fuels. Analysis of industry willingness and needs for transitioning from CO2 reuse to CO2 removal could ease the creation of onshore microalgae CDR pathways, but more research is necessary to determine if this is possible and under what conditions.
First-Order Priorities
Accelerate the design, permitting, and execution of the next generation of controlled field trials to answer questions specifically pertaining to carbon sequestration
- For open ocean systems, under various conditions
-
- Determine the effectiveness (including bioavailability) of nutrient delivery at supporting new primary production
- Determine the efficacy of export from the surface ocean into the deep ocean, and the associated estimates of carbon sequestration durability
- Determine the resulting air-sea fluxes of carbon dioxide and other greenhouse gases to quantify the additionality of the nutrient fertilization
- Characterize the environmental impacts, both intentional and unintentional, of any nutrient fertilization activity, including:
- Changes to upper ocean biology, chemistry, and physics (especially for artificial upwelling)
- Impacts to upper trophic levels and food web interactions
- Impacts to existing marine industries and resource needs (e.g., fisheries)
- For closed systems
- Demonstrate net negative carbon removal pathways in closed system cultivation
- Determine sequestration efficacy and durability from various carbon storage possibilities, including land-based burial, storage in the deep ocean, and storage on the seafloor
- Characterize the environmental impacts, both intentional and unintentional, of any cultivation and sequestration activity, including:
- Land-based impacts from onshore cultivation construction and operation
- Impacts from leakage or spill of onshore systems
- Impacts to other marine-based operations (e.g., fisheries)
Map optimal locations for microalgae-based approaches and engage with interested and affected constituents
- Perform siting analyses to identify optimal locations for microalgae CDR that consider the suite of technical, economic, social, and political factors necessary for deployment, as well as potential conflicts and co-benefits with other marine industries (e.g., fisheries, renewable energy, etc.)
Optimize and/or efficiently automate all approaches for scalability and economic feasibility
- Develop tools and instruments to increase harvest efficiency for microalgae grown in contained systems
- Determine and/or develop optimal microalgae strains for maximally efficient CO2 uptake for various environments and settings
Create and adopt of a code of conduct for responsible research
- There has yet to be widespread adoption of a single code of conduct for CDR research, development, and deployment, although some organizations have proposed guidelines for the development of such a code (see American Geophysical Union and the Aspen Institute) or have an internal code of conduct to which they adhere (see Planetary).
Create government frameworks that facilitate responsible field trials
- Clear frameworks that allow for responsible field trials of microalgae-based CDR to take place on land, in coastal waters, and in the open ocean are critical to accelerate progress. Without such frameworks there is risk of inappropriate experimental siting and delays in moving research out of the lab and into the real world. Climate interventions are time sensitive and responsible research, development, and deployment relies on comprehensive, cohesive, and effective government structures.
Blue Carbon Restoration and Carbon Sequestration
Introduction to the Road Map
Overview
The term “blue carbon” has historically referred to three (vascularly) vegetated coastal ecosystems: mangrove forests, salt marshes, and seagrass beds1. These ecosystems are among the most productive in the world2 and sequester carbon in their underlying substrates (e.g., soil or sediment). Estimates of their global distribution reach ~36-185 million hectares, storing as much as 33 billion tons of carbon3, however there remain large knowledge gaps around spatial extent and storage capacity, owing to the high variability across regions and ecosystems. The carbon in the substrate can be stored as organic or inorganic carbon and can remain sequestered for millennia4,5, positioning these ecosystems as important parts of the global carbon cycle. Situated between land and water, these ecosystems also play critical roles in human society and provide many services including coastal buffering from storms, surges and erosion control generally, nursery grounds for fish, culturally significant sites, and sources of income from fishing and tourism. Blue carbon ecosystems have gained attention as levers for climate change mitigation, including carbon sequestration6.
Some of this attention is related to the major uptick in interest and attention around carbon dioxide removal (CDR)7, which has again raised questions about the capacity of blue carbon ecosystems to sequester carbon and combat climate change8,9,10,11. This discussion has also broadened the conversation around the definition of blue carbon, with the IPCC (2019)12 defining blue carbon as ‘all biologically driven carbon fluxes and storage in marine systems that are amenable to management’. This definition can and has been interpreted to encompass not only coastal vegetated ecosystems that store carbon in their structure and sediments, but also other marine life such as fish and mammals, as well as natural seaweed stands and seaweed farms - all systems that not only store and recycle carbon through their living tissues, but also respond to anthropogenic manipulation and management. Two notable examples of this broadened definition include the National Academies of Sciences, Engineering, and Medicine’s (NASEM) 2022 report on ocean carbon dioxide removal in which they include a chapter on “Recovery of Marine Ecosystems” for CDR (including benthic, pelagic, and offshore ocean systems along with mangrove forests, salt marshes, and seagrass beds) and the National Oceanographic and Atmospheric Administration’s (NOAA) 2023 Strategy for Carbon Dioxide Removal Research which includes coastal blue carbon (defined as mangrove forests, salt marshes, and seagrass beds) as well as ‘Marine Ecosystem Recovery’ (fishes and marine mammals). (Also note NOAA, 2021.).
Following these recent advances, this road map will consider carbon stored in natural seaweed stands and in marine animals (e.g., fishes and mammals) in the definition of blue carbon, alongside mangrove forests, salt marshes, and seagrass beds. Natural seaweed stands and marine animals have received substantially less attention as potential pathways for additional carbon dioxide sequestration and will require accelerated research to determine their potential. The nascent nature of these pathways makes them particularly well suited to be assessed in this road map.
Note: While the blue carbon definition above could be interpreted to include microalgae cultivation, we have excluded it from consideration in this road map because we have already released a road map for Microalgae Cultivation and Carbon Sequestration. In addition, we limit discussion of seaweed (macroalgae) farms to coastal farms which passively sequester carbon through biomass export to underlying sediments or offshore to deeper waters. We exclude discussion of seaweed farming coupled to active sequestration (such as sinking or harvesting to produce biochar) because this topic is covered in a different road map, Macroalgae Cultivation and Carbon Sequestration.
This road map focuses on assessing the potential for blue carbon to contribute to carbon dioxide removal (CDR), or the additional sequestration of carbon, and what is needed to achieve that potential. In many cases, this will look like the large-scale restoration of ecosystems or rebuilding of animal populations (and, in some cases, expansion of ecosystems or animal populations beyond past limits). Recent work by the Environmental Defense Fund and the High Level Panel for a Sustainable Ocean Economy suggests that even the most rigorous and optimistic future estimates of blue carbon restoration may yield small gains in additional carbon sequestration when compared with the scale of anthropogenic emissions and other possible CDR pathways13,14,15,16. One reason for this is the spatial limitation presented by the restoration of coastal vegetated ecosystems (i.e., mangrove forests, seagrass beds, and salt marshes). These ecosystems have spatial limits on their potential for restoration, and thus, their potential for carbon removal, however other sources of blue carbon, such as animal biomass and seaweed farms, are far less limited by spatial constraints. Moreover, blue carbon restoration offers low or no risk pathways, which sets it apart from other proposed CDR solutions. Not only do restoration efforts of blue carbon pose fewer risks to the environment and society than other proposed CDR solutions, but they also offer an enormous capacity for other benefits to both ecosystems and communities. In this way, carbon removal may in fact be best thought of as a “co-benefit” to blue carbon restoration and efforts17. Regardless, there is still critical research that would increase our understanding of the role blue carbon plays in the carbon cycle and inform responsible decision making at the ocean-climate nexus.
In light of current and future climate disruption and risk, there is a growing need for solutions that will help to remove and durably store legacy greenhouse gas emissions. However, there remain a substantial set of unanswered questions around the capacity and efficacy of carbon storage in blue carbon systems. As scientists, practitioners, and policy makers continue investigating blue carbon as a climate solution, it is critical to be clear about what is known, what remains unknown, and identify a pathway by which to answer the most important outlying questions. This road map lays out the current state of knowledge around carbon sequestration and storage in blue carbon systems, details the most pressing unanswered questions to understand the potential for blue carbon sequestration, and proposes ways in which to accelerate the development and testing of these potential solution pathways.
A Note on Restoration vs. Protection
Protection of existing blue carbon ecosystems and their embedded carbon avoid emissions generated as these systems degrade. This is complementary and distinct from restoration of blue carbon systems, which is regrowth of these ecosystems. This regrowth is where additional carbon can be sequestered. Because the focus of this road map is the potential for blue carbon systems to contribute to carbon dioxide removal, this map will only focus on restoration efforts, their state of progress, challenges, knowledge gaps, and opportunities to drive forward progress.
A Note on Units of Measurement
This road map differs from the other Ocean Visions’ Ocean CDR Road Maps in its use of ‘Gigaton CO2 equivalent’ (Gt CO2e) in order to account for the important role played by non-CO2 greenhouse gases (e.g., methane, CH4, and nitrous oxide, N2O) within blue carbon systems. Although petagrams (Pg) is a unit commonly used in blue carbon literature, we use the equivalent gigatons (or gigatonnes; Gt) in this road map.
The Environmental Defense Fund published three detailed reports in 2022 on "Natural Climate Solutions" that examine the roles that coastal blue carbon, natural seaweed stands, and marine animals may play in the overall climate solutions landscape, including, but not limited to, carbon removal. For more in-depth reading about any one of these systems and the other roles they can play beyond carbon removal, we recommend exploring the recent suite of detailed reports (linked below) as well as the many cited papers throughout this road map. There are also several other resources embedded in this road map including links to blue carbon working groups around the globe where current research and work is happening and interactive maps showing known extents of blue carbon habitats.
|
- Lovelock CE, Duarte CM. 2019 Dimensions of Blue Carbon and emerging perspectives. Biol. Lett. 15: 20180781. http://dx.doi.org/10.1098/rsbl.2018.0781
- National Academies of Sciences, Engineering, and Medicine 2019. Negative Emissions Technologies and Reliable Sequestration: A Research Agenda. Washington, DC: The National Academies Press. https://doi.org/10.17226/25259.
- Macreadie, P. I., Costa, M. D. P., Atwood, T. B., Friess, D. A., Kelleway, J. J., Kennedy, H., ... Duarte, C. M. (2021). Blue carbon as a natural climate solution. Nature Reviews Earth & Environment. doi:10.1038/s43017-021-00224-1
- Macreadie, P. I., Costa, M. D. P., Atwood, T. B., Friess, D. A., Kelleway, J. J., Kennedy, H., ... Duarte, C. M. (2021). Blue carbon as a natural climate solution. Nature Reviews Earth & Environment. doi:10.1038/s43017-021-00224-1
- Duarte, C. M., Losada, I. J., Hendriks, I. E., Mazarrasa, I. & Marba, N. 2013. The role of coastal plant communities for climate change mitigation and adaptation. Nat. Clim. Chang. 3:961–8.
- Collins, J. R., Boenish, R. E., Kleisner, K. M., Rader, D. N., Fujita, R. M., and Moritsch M. M. 2022. Coastal natural climate solutions: an assessment of scientific knowledge surrounding pathways for carbon dioxide removal and avoided emissions in nearshore blue carbon ecosystems. Environmental Defense Fund, New York, NY. https://www.edf.org/sites/default/files/2022-10/Coastal%20Natural%20Climate%20Solutions.pdf
- “Anthropogenic activities removing CO2 from the atmosphere and durably storing it in geological, terrestrial, or ocean reservoirs, or in products. It includes existing and potential anthropogenic enhancement of biological or geochemical sinks and direct air capture and storage, but excludes natural CO2 uptake not directly caused by human activities.” https://www.ipcc.ch/sr15/chapter/glossary/
- Collins, J. R., Boenish, R. E., Fujita, R. M., Rader, D. N., and Moore, L. A. 2022. Natural climate solutions in the open ocean: scientific knowledge and opportunities surrounding four potential pathways for carbon dioxide removal or avoided emissions. Environmental Defense Fund, New York, NY. https://www.edf.org/sites/default/files/2022-10/Natural%20Climate%20Solutions%20in%20the%20Open%20Ocean.pdf
- National Academies of Sciences, Engineering, and Medicine 2022. A Research Strategy for Ocean-based Carbon Dioxide Removal and Sequestration. Washington, DC: The National Academies Press. https://doi.org/10.17226/26278.
- Cross, J.N., Sweeney, C., Jewett, E.B., Feely, R.A., McElhany, P., Carter, B., Stein, T., Kitch, G.D., and Gledhill, D.K., 2023. Strategy for NOAA Carbon Dioxide Removal Research: A white paper documenting a potential NOAA CDR Science Strategy as an element of NOAA’s Climate Interventions Portfolio. NOAA Special Report. NOAA, Washington DC. DOI: 10.25923/gzke-8730
- National Academies of Sciences, Engineering, and Medicine 2019. Negative Emissions Technologies and Reliable Sequestration: A Research Agenda. Washington, DC: The National Academies Press. https://doi.org/10.17226/25259.
- IPCC, 2019: Annex I: Glossary [Weyer, N.M. (ed.)]. In: IPCC Special Report on the Ocean and Cryosphere in a Changing Climate [H.-O. Pörtner, D.C. Roberts, V. Masson-Delmotte, P. Zhai, M. Tignor, E. Poloczanska, K. Mintenbeck, A. Alegría, M. Nicolai, A. Okem, J. Petzold, B. Rama, N.M. Weyer (eds.)]. Cambridge University Press, Cambridge, UK and New York, NY, USA, pp. 677–702. https://doi.org/10.1017/9781009157964.010.
- Fujita, R. M., Collins, J. R., Kleisner, K. M., Rader, D. N., Mejaes, A., Augyte, S., and Brittingham, P. A., 2022. Carbon sequestration by seaweed: background paper for the Bezos Earth Fund - EDF workshop on seaweed carbon sequestration. Environmental Defense Fund, New York, NY. https://www.edf.org/sites/default/files/2022-10/Carbon%20Sequestration%20by%20Seaweed.pdf
- Collins, J. R., Boenish, R. E., Kleisner, K. M., Rader, D. N., Fujita, R. M., and Moritsch M. M. 2022. Coastal natural climate solutions: an assessment of scientific knowledge surrounding pathways for carbon dioxide removal and avoided emissions in nearshore blue carbon ecosystems. Environmental Defense Fund, New York, NY. http://www.edf.org/sites/default/files/2022-10/Coastal%20Natural%20Climate%20Solutions.pdf
- Collins, J. R., Boenish, R. E., Fujita, R. M., Rader, D. N., and Moore, L. A. 2022. Natural climate solutions in the open ocean: scientific knowledge and opportunities surrounding four potential pathways for carbon dioxide removal or avoided emissions. Environmental Defense Fund, New York, NY. https://www.edf.org/sites/default/files/2022-10/Natural%20Climate%20Solutions%20in%20the%20Open%20Ocean.pdf
- Hoegh-Guldberg, O., Northrop, E. et al. 2023. "The ocean as a solution to climate change: Updated opportunities for action." Special Report. Washington, DC: World Resources Institute. Available online at https://oceanpanel.org/publication/ocean-solutions-to-climate-change
- Williamson P and Gattuso J-P (2022) Carbon Removal Using Coastal Blue Carbon Ecosystems Is Uncertain and Unreliable, With Questionable Climatic Cost-Effectiveness. Front. Clim. 4:853666. doi: 10.3389/fclim.2022.853666
Summary Figures
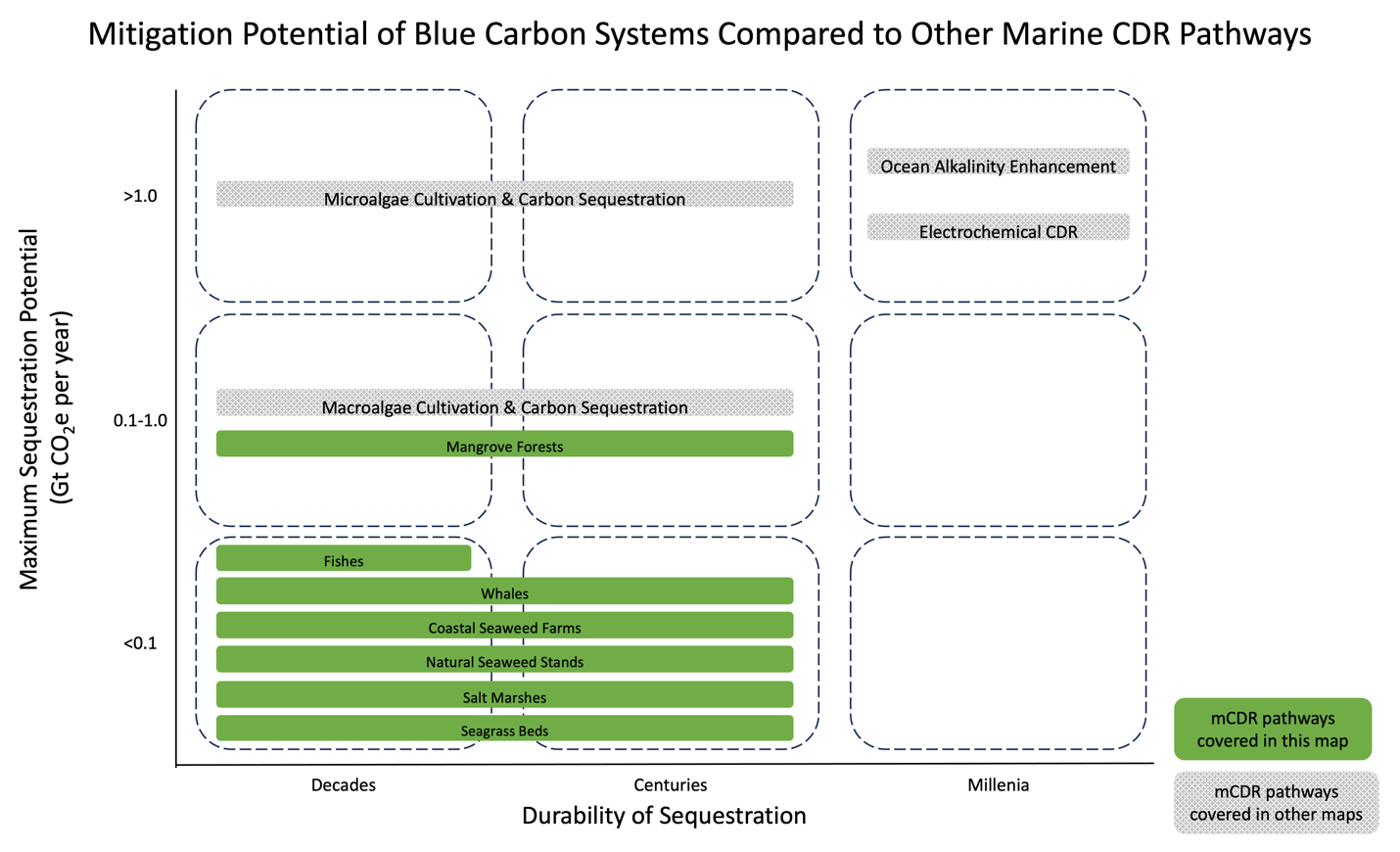
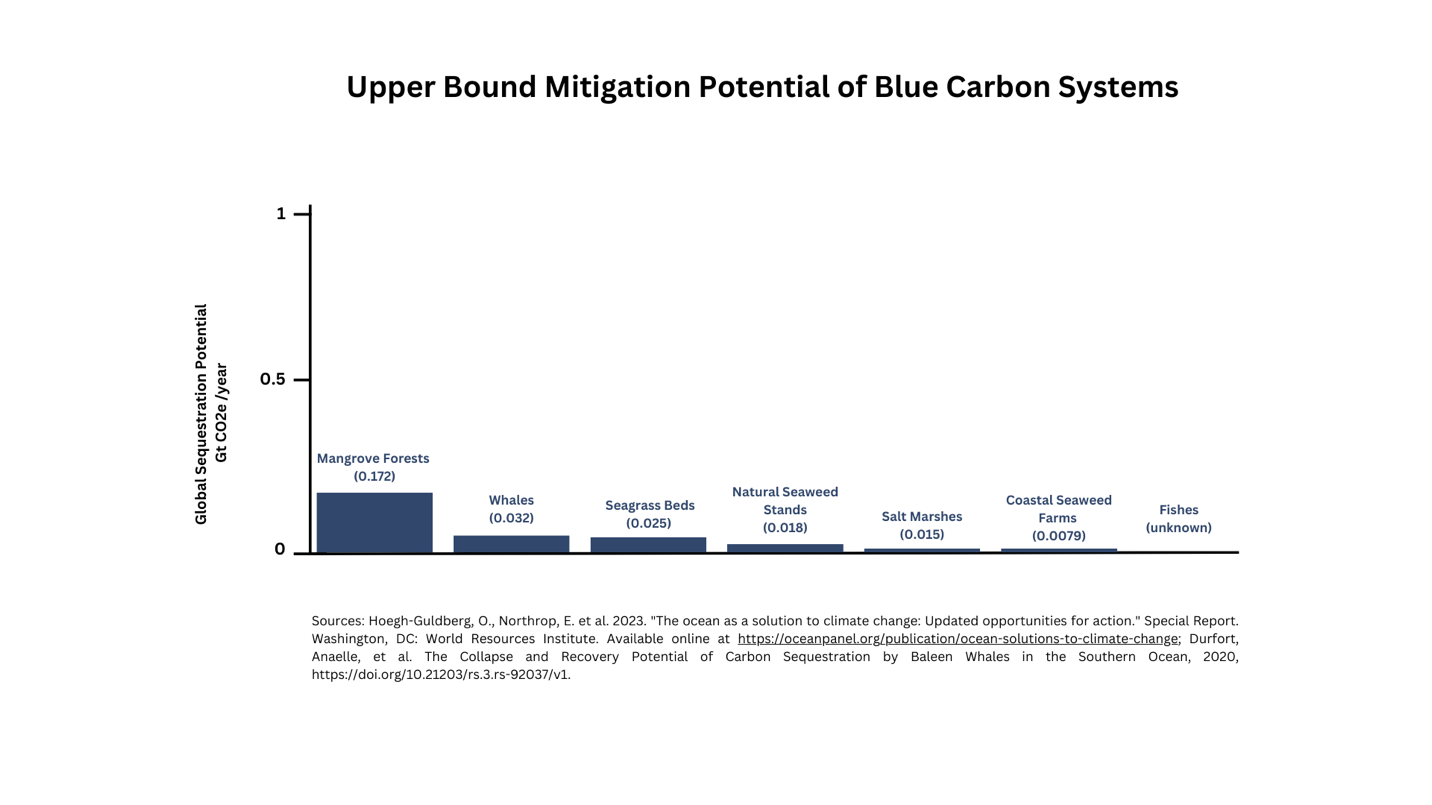
Visual of Environmental Co-Benefits Across Blue Carbon Systems
Environmental Co-Benefits
|
|||||||||||
Co-Benefit |
System |
||||||||||
Animal Carbon |
Natural Seaweed Stands |
Mangrove Forests |
Salt Marshes |
Seagrass Beds |
|||||||
Amelioration of ocean acidification |
(Mongin, 2016) |
(Banjeree & Paul, 2022) |
(Ricart et al., 2022; Koweek et al. 2018) |
||||||||
Potential for ocean alkalinity enhancement |
(Fakhraee et al., 2023) |
(Fakhraee et al., 2023) |
|||||||||
Contribution to biodiversity |
(Theuerkauf et al., 2021) |
(Thrush et al., 2013; Esteves 2014) |
(McHenry et al. 2021) |
||||||||
Contribution to habitat provisioning |
(Langton et al., 2019) |
||||||||||
Contribution to heavy metal and nutrient pollution removal |
(Zheng et al, 2019; Jiang et al., 2020) |
(Lee et al. 2019) |
|||||||||
Contribution to fishery enhancement |
(Rimmer et al., 2021; Theuerkauf et al., 2021) |
(Orth et al., 2006) |
|||||||||
Improved water clarity through the facilitation of settlement of fine sediments |
(Jiang et al., 2020) |
||||||||||
Coastal protection against erosion and/or sea level rise |
(Elsmore et al., 2023) |
(Barbier et al., 2011) |
(Barbier et al., 2011) |
(Barbier et al., 2011, Duarte et al. 2013) |
|||||||
Nutrient cycling |
(Le Mézo et al. 2022; Ratnarajah et al., 2014)
|
(Cotas et al., 2023)
|
(Mitra 2019) |
(Sousa et al., 2010) |
(Orth et al., 2006) |
||||||
Reducing biodiversity loss |
(NASEM 2022 mCDR) |
(NASEM 2022 mCDR) |
(NASEM 2022 mCDR) |
(NASEM 2022 mCDR) |
|||||||
Restoring the role of marine organisms in the carbon cycle |
(NASEM 2022 mCDR) |
(NASEM 2022 mCDR) |
(NASEM 2022 mCDR) |
(NASEM 2022 mCDR) |
(NASEM 2022 mCDR) |
Coastal Vegetated Ecosystems: Mangrove Forests, Salt Marshes, and Seagrass Beds
Overview
Mangrove forests, salt marshes, and seagrass beds grow where land meets the sea and store carbon in their vegetation and underlying sediments/soils. The sediments/soils can store both organic and inorganic carbon. Some of this carbon originates from within the ecosystem itself (autochthonous carbon) while some originates from outside the ecosystem (allochthonous carbon). The exact proportion of autochthonous versus allochthonous carbon is difficult to determine, and differs by site, but is important when trying to account for all the carbon fluxes in a system. What’s more, carbon can move laterally and vertically in, out, and through these systems, creating additional complexity for carbon accounting.
Of these three ecosystems, in terms of current global extent, current carbon storage, and carbon sequestration potential, mangrove forests are the best understood followed by salt marshes. Seagrass beds are the least well understood both in spatial extent and ultimate carbon sequestration potential.
Restoration and its limitations: CDR pathways within coastal vegetated ecosystems are primarily concerned with restoration – the act of bringing an ecosystem back as nearly as possible to its original condition1. However, the goal of restoration may be unattainable for several reasons, including irreversible land and hydrological (starved of freshwater input) change, conditions that do not permit rehabilitation, and the inherent spatial limitations for ecosystems that occupy specific niches2. Another challenge is the host of known and unknown consequences of climate change which may impact the feasibility and efficacy of restoration efforts. Monitoring of restoration projects will also be critical both as important data points for studies and predictive science as well as for any involvement with carbon markets. There has been historical reluctance from both government agencies and private developers to fund long-term monitoring3, however this will be crucial for informed decision making about where and how to implement restoration projects.
Net sequestration of CO2e and its storage durability are key metrics when considering restoration projects for CDR. Ideally, monitoring of restoration projects should feed back into adaptive management practices, where management techniques are adjusted though an iterative process in response to other variables such as climate change.
- As defined by the US National Research Council (1992)
- Adam, Paul. “Salt Marsh Restoration.” Coastal Wetlands, 2019, pp. 817–861, https://doi.org/10.1016/b978-0-444-63893-9.00023-x.
- Adam, Paul. “Salt Marsh Restoration.” Coastal Wetlands, 2019, pp. 817–861, https://doi.org/10.1016/b978-0-444-63893-9.00023-x.
Mangrove Forests
Mechanism for CDR
- Restoring hydrology: Restoration of hydrology, through reversing drainage or removing tidal restrictions may assist in the recovery and expansion of existing mangrove sites. This path may be met with more resistance (especially political and social) than other types of restoration efforts.
- Planting mangrove seedlings: This is one of the most common restoration techniques, however, large-scale planting efforts have often failed1, (many of these shortcomings a result of projects that do not adequately consider environmental and socioeconomic conditions2) and smaller projects may not deliver carbon storage on climate-relevant scales3.
-
- Research is being done on the efficacy of automated mangrove planting via drones4,5. This work is still in early stages but may provide lower cost options for planting efforts, especially in difficult to access locations.
- Restoration efforts should be de-risked in smaller projects before moving to larger projects. This will require investment in capacity-building in communities and institutions, and mechanisms to match restoration opportunities with prospective supporters and investors6.
CDR Potential
Estimated Sequestration Potential: 0.028 – 0.172 Gt CO2e/year7
Sequestration Durability: Decades -- 1,000 years8 (Durability of sequestered carbon will depend on multiple variables and can vary greatly between individual ecosystems)
Challenges
- Accounting:
-
- Estimating future sequestration potentials or future rates of carbon sequestration is more difficult than estimating their current global extent and storage.
- While there is general scientific consensus that mangroves are net sinks of CO29, there is growing research that indicates production of CH4 and N2O nonuniformly across habitats. In order to have accurate calculations of greenhouse gas budgets, these emissions will need to be included in the accounting10.
- Current estimates of sequestration potential do not account for the effects of climate change on the ecosystem (e.g., warming, sea level rise, coastal squeeze, increased tropical storms).
- Mangroves are inextricably connected to other marine ecosystems like seagrasses and coral reefs, complicating the definition of boundaries for estimating “additional” carbon sequestration and introducing other complications, such as figuring out how much carbon is autochthonous and how much is allochthonous. Additionally, many carbon markets currently require that all carbon be autochthonous to count toward credits or have poorly defined frameworks for whether to credit for allochthonous carbon that accumulates on site as a result of restoration or management actions.
- Restoration:
-
- There are high costs associated with restoration efforts11.
- Mangrove ecosystems are heterogeneous across geographies, and this must be accounted for when assessing individual habitat potential for carbon sequestration. (e.g., findings from one location/ecosystem may not be directly applicable to other location/ecosystems
- There is work being done to use unmanned aerial systems (UAS) to assess mangrove estuaries on the Pacific Coast of Costa Rica12 and in Malaysia13, enabling monitoring of difficult to access locations. This type of innovation may be important for monitoring and verification of restored mangrove forests.
Key Knowledge Gaps
- Quantitative understanding of the impacts of climate change on mangrove ecosystems and how these may affect restoration efforts.
- Incomplete understanding of greenhouse gas production and release (e.g., CH4 and N2O) by and from mangroves and how this impacts net carbon removal.
- Uncertainty around durability of carbon stored in mangroves and their sediments as well as carbon burial rates14.
First-Order Priorities
*Adapted from the Environmental Defense Fund report on Coastal Natural Climate Solutions15
- Improve predictions of the effects of climate change on mangrove forests*
- Predictions needed include:
- Effects of increased tropical storm intensity and frequency on the destruction and degradation of mangrove ecosystems
- The timescales over which any previously sequestered carbon could be released to the atmosphere
- Understand/map the potential spatial extent of mangrove forests given climate change impacts on existing habitats and poleward migration of mangroves with future warming.
- Predictions needed include:
- Improved, spatially explicit modeling approaches to predict greenhouse gas fluxes and net carbon sequestration*
- Models should function across different mangrove ecosystems whose sediment dynamics cannot be resolved solely using imagery taken from space or aircraft.
- The development of carbon crediting projects requires we know not only how much carbon a mangrove ecosystem contains in its above and belowground biomass, but also the magnitude of various greenhouse gas fluxes from underlying soils or sediments at present and in the future.
- These models should account for future ecosystem state due to the interactive effects of climate change and predicted land-use changes, including the phenomenon of coastal squeeze, the prevention of expansion due to rural areas on one side and the ocean on the other.
- Create studies designed to better differentiate between carbon originating from within the system (autochthonous) and carbon originating from elsewhere (allochthonous)*
- The ability to distinguish among carbon sources is particularly critical since methodologies developed under the voluntary carbon market standards require deduction of allochthonous carbon from any claimed credit.
- Accelerate the development of technology and biotechnology that can help scale restoration efforts
- Optimize drones and autonomous robots for increased scalability of mangrove planting.
- Test biological engineering techniques to enhance carbon storage within mangrove forests.
- Zimmer M, Ajonina GN, Amir AA, Cragg SM, Crooks S, Dahdouh-Guebas F, Duke NC, Fratini S, Friess DA, Helfer V, Huxham M, Kathiresan K, Kodikara KAS, Koedam N, Lee SY, Mangora MM, Primavera J, Satyanarayana B, Yong JWH and Wodehouse D (2022) When nature needs a helping hand: Different levels of human intervention for mangrove (re-)establishment. Front. For. Glob. Change 5:784322. doi: 10.3389/ffgc.2022.784322
- Lovelock CE,Barbier E,Duarte CM(2022) Tackling the mangrove restoration challenge. PLoS Biol20(10): e3001836. https://doi.org/10.1371/journal.pbio.3001836
- Lovelock CE, Barbier E, Duarte CM (2022) Tackling the mangrove restoration challenge. PLoS Biol 20(10): e3001836. https://doi.org/10.1371/journal.pbio.3001836
- AlRaisi, A. A., et al. “Restoration technology hand in hand with nature-based solutions: ADNOC’s drone led Mangrove Restoration Project.” Day 1 Mon, October 02, 2023, 2023, https://doi.org/10.2118/215963-ms.
- Ayub, Ayub Sugara, et al. “Mapping the potential of mangrove planting in the rehabilitation of coastal ecosystems using drone technology.” Journal of Sylva Indonesiana, vol. 6, no. 02, 2023, pp. 164–177, https://doi.org/10.32734/jsi.v6i02.10515.
- Lovelock CE, Barbier E, Duarte CM (2022) Tackling the mangrove restoration challenge. PLoS Biol 20(10): e3001836. https://doi.org/10.1371/journal.pbio.3001836
- Hoegh-Guldberg, O., Northrop, E. et al. 2023. "The ocean as a solution to climate change: Updated opportunities for action." Special Report. Washington, DC: World Resources Institute. Available online at https://oceanpanel.org/publication/ocean-solutions-to-climate-change
- Cross, J.N., Sweeney, C., Jewett, E.B., Feely, R.A., McElhany, P., Carter, B., Stein, T., Kitch, G.D., and Gledhill, D.K., 2023. Strategy for NOAA Carbon Dioxide Removal Research: A white paper documenting a potential NOAA CDR Science Strategy as an element of NOAA’s Climate Interventions Portfolio. NOAA Special Report. NOAA, Washington DC. DOI: 10.25923/gzke-8730
- Rosentreter, Judith A., et al. “Coastal vegetation and estuaries are collectively a greenhouse gas sink.” Nature Climate Change, vol. 13, no. 6, 2023, pp. 579–587, https://doi.org/10.1038/s41558-023-01682-9.
- Collins, J. R., Boenish, R. E., Kleisner, K. M., Rader, D. N., Fujita, R. M., and Moritsch M. M. 2022. Coastal natural climate solutions: an assessment of scientific knowledge surrounding pathways for carbon dioxide removal and avoided emissions in nearshore blue carbon ecosystems. Environmental Defense Fund, New York, NY. https://www.edf.org/sites/default/files/2022-10/Coastal%20Natural%20Climate%20Solutions.pdf
- Vanderklift M, Steven A, Marcos-Martinez R, Gorman D (2018) Achieving carbon offsets through blue carbon: a review of needs and opportunities relevant to the Australian seafood industry, FRDC Final Report 2018/060. CSIRO Oceans and Atmosphere, Crawley, November 2018.
- Yaney-Keller A, Santidrián Tomillo P, Marshall JM, Paladino FV (2019) Using Unmanned Aerial Systems (UAS) to assay mangrove estuaries on the Pacific coast of Costa Rica. PLoS ONE 14(6): e0217310. https://doi.org/10.1371/journal.pone.0217310
- Otero, Viviana, et al. “Managing mangrove forests from the Sky: Forest Inventory using field data and Unmanned Aerial Vehicle (UAV) imagery in the Matang Mangrove Forest Reserve, peninsular Malaysia.” Forest Ecology and Management, vol. 411, 2018, pp. 35–45, https://doi.org/10.1016/j.foreco.2017.12.049.
- Williamson P and Gattuso J-P (2022) Carbon Removal Using Coastal Blue Carbon Ecosystems Is Uncertain and Unreliable, With Questionable Climatic Cost-Effectiveness. Front. Clim. 4:853666. doi: 10.3389/fclim.2022.853666
- Collins, J. R., Boenish, R. E., Kleisner, K. M., Rader, D. N., Fujita, R. M., and Moritsch M. M. 2022. Coastal natural climate solutions: an assessment of scientific knowledge surrounding pathways for carbon dioxide removal and avoided emissions in nearshore blue carbon ecosystems. Environmental Defense Fund, New York, NY. https://www.edf.org/sites/default/files/2022-10/Coastal%20Natural%20Climate%20Solutions.pdf
Salt Marshes
Mechanism for CDR
- Most restoration efforts include recovery of tidal exchange (e.g., restoring hydrology and tidal flow), managed realignment, and sediment level amendment1. Efforts can also include removal of non-native vegetation and/or replanting of native vegetation.
- For an in-depth look at salt marsh restoration efforts to date, refer to Adam 2019 “Salt Marsh Restoration”2
CDR Potential
Estimated Sequestration Potential: 0.004 – 0.015 Gt CO2e/year3
Sequestration Durability: Decades -- 1,000 years4 (Durability of sequestered carbon will depend on multiple variables and can vary greatly between individual ecosystems)
Challenges
- Little potential for expansion of current habitats due to extensive land conversion.
- Gaining access to suitable land for restoration projects may be difficult and expensive.
- Determining baseline historical extent for setting restoration objectives.
Key Knowledge Gaps
- Need accurate predictions of carbon sequestration which requires integrated modeling of ecological impacts, land-use, and climate effects (made more challenging by high variability in carbon burial rates5).
- Discerning between competing feedbacks associated with climate and sea level rise, which can have both positive and negative impacts on ecosystems.
- Measurement of greenhouse gases other than CO2 (e.g., CH4 and N2O) that are produced and their significance to net sequestration.
- What is the fate of carbon produced outside of marshes and trapped in them?
- Total global areal existing extent remains poorly mapped, especially at high latitudes.
First-Order Priorities
*Adapted from the Environmental Defense Fund report on Coastal Natural Climate Solutions6
- Conduct research to better understand and characterize the multiple carbon fluxes within salt marsh systems
- Improve predictions of carbon sequestered by salt marshes through modeling*
- Better knowledge of the relative contributions of carbon produced outside of (allochthonous) and within (autochthonous) marshes to salt marsh organic carbon pools, and the labilities of the carbon derived from these two sources*
- Investigate and characterize climate impacts, both positive and negative, on salt marsh systems*
- Sea level rise, warming, and increased coastal storm frequency and severity all need further investigation.
- Measure non-CO2 greenhouse gases that are produced and compare with sequestration capabilities to better understand the net sequestration of salt marshes*
- Better global mapping of the current extent of salt marshes, particularly at high latitudes, as well as habitat-suitability mapping/analysis*
- Billah, M., Bhuiyan, M.A., Islam, M.A. et al. Salt marsh restoration: an overview of techniques and success indicators. Environ Sci Pollut Res 29, 15347–15363 (2022). https://doi.org/10.1007/s11356-021-18305-5
- Adam, Paul. “Salt Marsh Restoration.” Coastal Wetlands, 2019, pp. 817–861, https://doi.org/10.1016/b978-0-444-63893-9.00023-x.
- Hoegh-Guldberg, O., Northrop, E. et al. 2023. "The ocean as a solution to climate change: Updated opportunities for action." Special Report. Washington, DC: World Resources Institute. Available online at https://oceanpanel.org/publication/ocean-solutions-to-climate-change
- Cross, J.N., Sweeney, C., Jewett, E.B., Feely, R.A., McElhany, P., Carter, B., Stein, T., Kitch, G.D., and Gledhill, D.K., 2023. Strategy for NOAA Carbon Dioxide Removal Research: A white paper documenting a potential NOAA CDR Science Strategy as an element of NOAA’s Climate Interventions Portfolio. NOAA Special Report. NOAA, Washington DC. DOI: 10.25923/gzke-8730
- Williamson P and Gattuso J-P (2022) Carbon Removal Using Coastal Blue Carbon Ecosystems Is Uncertain and Unreliable, With Questionable Climatic Cost-Effectiveness. Front. Clim. 4:853666. doi: 10.3389/fclim.2022.853666
- Collins, J. R., Boenish, R. E., Kleisner, K. M., Rader, D. N., Fujita, R. M., and Moritsch M. M. 2022. Coastal natural climate solutions: an assessment of scientific knowledge surrounding pathways for carbon dioxide removal and avoided emissions in nearshore blue carbon ecosystems. Environmental Defense Fund, New York, NY. https://www.edf.org/sites/default/files/2022-10/Coastal%20Natural%20Climate%20Solutions.pdf
Seagrass Beds
Mechanisms for CDR
- Unlike salt marshes and mangrove forests, seagrass beds remove carbon from seawater, creating a disequilibrium in the CO2 concentration between the seawater and atmosphere. The resulting flux from the atmosphere into the seawater replenishes the deficit in dissolved inorganic carbon due to seagrass photosynthesis. This replenishment may be complete (which maximizes carbon removal) or incomplete (which results in an inefficiency in carbon removal accounting).
- In theory, restoration (e.g., seed/seedling dispersal or planting) is the most likely mechanism for CDR, however, efforts to date are often unsuccessful1. Restoration propositions are further complicated by the outstanding questions around net carbon removal (especially under different climate change scenarios) and the extent of suitable locations.
- A 2023 study in Australia found that sediment carbon stocks show little recovery immediately after restoration and can take >5 years to return to expected carbon sequestration rates2.
- Restoration of hydrology, through reversing drainage or removing tidal restrictions, may assist in the recovery and expansion of existing seagrass beds3. This path may be met with more resistance (especially political and social) than other types of restoration efforts.
CDR Potential
Estimated Sequestration Potential: 0.007 – 0.025 Gt CO2e/year4
Sequestration Durability: Decades -- 1,000 years5 (Durability of sequestered carbon will depend on multiple variables and can vary greatly between individual ecosystems)
Challenges
- A 2023 review of all available literature on eelgrass (the dominant species of seagrass along the United States west coast) restoration projects in California, Oregon, and Washington found that of the 82 restoration projects, only 6 were published in peer-reviewed journals. This highlights the lack of data availability that can make assessing successes, failures, and best practices difficult6.
- Difficult to differentiate between autochthonous and allochthonous sources of carbon in sediments7
- There is substantial inorganic carbon in seagrasses which is derived from outside the seagrass bed8 which in turn has sparked debate over whether seagrasses are ultimately a carbon sink or source.
- Seagrass restoration efforts are often unsuccessful9
- High variability in carbon burial rates10
- High cost associated with restoration efforts11
Key Knowledge Gaps
- Robust measurements of air-water CO2 exchange above seagrass beds
- Global extent of seagrasses (including future extent under changing climate)
- Causes of failed restoration projects
- What are the sources and fates of carbonates in seagrass beds?
First-Order Priorities
*Adapted from the Environmental Defense Fund report on Coastal Natural Climate Solutions12
- Take additional measurements of air-sea gas exchange above seagrass beds*
- This will lead to the development of more robust predictive models to better understand CDR efficacy.
- Map the global extent of seagrasses and their likely future extent*
- This is likely to involve some combination of active remote sensing and field surveys as well as habitat suitability analyses.
- Mapping of likely future extent will involve considerations of future climate change impacts.
- Continue investigations into the causes of restoration project failures in seagrass systems*
- This effort will likely involve better monitoring, recording, and publication of data on restoration efforts.
- Improve the understanding of the sources and fate of carbonates in seagrass bed sediments*
- This includes the contributions to CO2 production both within seagrass beds and upstream of these ecosystems.
- Macreadie, P.I., Costa, M.D.P., Atwood, T.B. et al. Blue carbon as a natural climate solution. Nat Rev Earth Environ 2, 826–839 (2021). https://doi.org/10.1038/s43017-021-00224-1
- Rahayu, Yusmiana P., et al. “Little change in surface sediment carbon stock following seagrass restoration in Shark Bay, Western Australia.” Estuarine, Coastal and Shelf Science, vol. 294, 2023, p. 108535, https://doi.org/10.1016/j.ecss.2023.108535.
- National Academies of Sciences, Engineering, and Medicine 2019. Negative Emissions Technologies and Reliable Sequestration: A Research Agenda. Washington, DC: The National Academies Press. https://doi.org/10.17226/25259.
- Hoegh-Guldberg, O., Northrop, E. et al. 2023. "The ocean as a solution to climate change: Updated opportunities for action." Special Report. Washington, DC: World Resources Institute. Available online at https://oceanpanel.org/publication/ocean-solutions-to-climate-change
- Cross, J.N., Sweeney, C., Jewett, E.B., Feely, R.A., McElhany, P., Carter, B., Stein, T., Kitch, G.D., and Gledhill, D.K., 2023. Strategy for NOAA Carbon Dioxide Removal Research: A white paper documenting a potential NOAA CDR Science Strategy as an element of NOAA’s Climate Interventions Portfolio. NOAA Special Report. NOAA, Washington DC. DOI: 10.25923/gzke-8730
- Ward, Melissa, and Kathryn Beheshti. “Lessons learned from over thirty years of eelgrass restoration on the US West Coast.” Ecosphere, vol. 14, no. 8, 2023, https://doi.org/10.1002/ecs2.4642.
- Macreadie, P.I., Costa, M.D.P., Atwood, T.B. et al. Blue carbon as a natural climate solution. Nat Rev Earth Environ 2, 826–839 (2021). https://doi.org/10.1038/s43017-021-00224-1
- Saderne, V., Geraldi, N.R., Macreadie, P.I. et al. Role of carbonate burial in Blue Carbon budgets. Nat Commun 10, 1106 (2019). https://doi.org/10.1038/s41467-019-08842-6
- Macreadie, P.I., Costa, M.D.P., Atwood, T.B. et al. Blue carbon as a natural climate solution. Nat Rev Earth Environ 2, 826–839 (2021). https://doi.org/10.1038/s43017-021-00224-1
- Williamson P and Gattuso J-P (2022) Carbon Removal Using Coastal Blue Carbon Ecosystems Is Uncertain and Unreliable, With Questionable Climatic Cost-Effectiveness. Front. Clim. 4:853666. doi: 10.3389/fclim.2022.853666
- Vanderklift M, Steven A, Marcos-Martinez R, Gorman D (2018) Achieving carbon offsets through blue carbon: a review of needs and opportunities relevant to the Australian seafood industry, FRDC Final Report 2018/060. CSIRO Oceans and Atmosphere, Crawley, November 2018.
- Collins, J. R., Boenish, R. E., Kleisner, K. M., Rader, D. N., Fujita, R. M., and Moritsch M. M. 2022. Coastal natural climate solutions: an assessment of scientific knowledge surrounding pathways for carbon dioxide removal and avoided emissions in nearshore blue carbon ecosystems. Environmental Defense Fund, New York, NY. https://www.edf.org/sites/default/files/2022-10/Coastal%20Natural%20Climate%20Solutions.pdf
Environmental Co-Benefits of Coastal Vegetated Ecosystems
Restoration of coastal vegetated ecosystems can generate many benefits for surrounding marine ecosystems. While we are calling these “co-benefits” here, it should be noted that some of these benefits are so significant (while carbon sequestration feasibility and efficacy remain unknown) that these may in fact be the main benefits of restoration. (Note that there are significantly more co-benefits in coastal vegetated ecosystems than any of the other Ocean Visions’ marine CDR Road Maps.)
- Potential for ocean alkalinity enhancement1
- Coastal protection against erosion2
- Nutrient cycling3
- Pollutant filtering
- Reducing biodiversity loss4
- Restoring the role of marine organisms in the carbon cycle5
- Protection against storm surge and inundation6,7,8
- Providing habitat and nurseries for a variety of marine organisms9
- Fakhraee, Mojtaba, et al. “Ocean Alkalinity Enhancement through Restoration of Blue Carbon Ecosystems.” Nature Sustainability, 2023, https://doi.org/10.1038/s41893-023-01128-2.
- Barbier, E. B. et al. The value of estuarine and coastal ecosystem services. Ecol. 697 Monogr. 81, 169–193 (2011).
- Mitra, Abhijit. “Ecosystem Services of mangroves: An overview.” Mangrove Forests in India, 2019, pp. 1–32, https://doi.org/10.1007/978-3-030-20595-9_1.
- National Academies of Sciences, Engineering, and Medicine 2022. A Research Strategy for Ocean-based Carbon Dioxide Removal and Sequestration. Washington, DC: The National Academies Press. https://doi.org/10.17226/26278.
- National Academies of Sciences, Engineering, and Medicine 2022. A Research Strategy for Ocean-based Carbon Dioxide Removal and Sequestration. Washington, DC: The National Academies Press. https://doi.org/10.17226/26278.
- Blankespoor, B., Dasgupta, S. & Lange, GM. Mangroves as a protection from storm surges in a changing climate. Ambio 46, 478–491 (2017). https://doi.org/10.1007/s13280-016-0838-x
- Liu, Huiqing, et al. “Numerical study of the sensitivity of mangroves in reducing storm surge and flooding to hurricane characteristics in southern Florida.” Continental Shelf Research, vol. 64, 2013, pp. 51–65, https://doi.org/10.1016/j.csr.2013.05.015.
- Zhang, Keqi, et al. “The role of mangroves in attenuating storm surges.” Estuarine, Coastal and Shelf Science, vol. 102–103, 2012, pp. 11–23, https://doi.org/10.1016/j.ecss.2012.02.021.
- Whitfield, A.K. The role of seagrass meadows, mangrove forests, salt marshes and reed beds as nursery areas and food sources for fishes in estuaries. Rev Fish Biol Fisheries 27, 75–110 (2017). https://doi.org/10.1007/s11160-016-9454-x
Environmental Risks of Coastal Vegetated Ecosystems
Social Co-Benefits of Coastal Vegetated Ecosystems
Restoration of coastal vegetated ecosystems can generate many benefits for surrounding communities and economies. While we are calling these “co-benefits” here, it should be noted that some of these benefits are so significant (while carbon sequestration feasibility and efficacy remain unknown) that these may in fact be the main benefits of restoration. Many of the environmental benefits relate directly to social benefits, such as fisheries enhancement and coastal protection. (Note that there are significantly more co-benefits in coastal vegetated ecosystems than any of the other Ocean Visions’ marine CDR Road Maps)
- Coastal protection from storms, floods, and sea-level rise123
- Increased adaptive capacity for communities to handle climate change and natural disasters
- Job creation and economic stimulation from enhanced fisheries and tourism (of particular value to coastal communities that are dependent on marine-based livelihoods)
- Cultural / intrinsic / recreational value
- Education and research – the pursuit of restoring blue carbon for CDR will naturally add value to the existing body of research and can provide valuable educational experiences for the upcoming generation of scientists and conservationists.
- Kiesel, J. et al. Effective design of managed realignment schemes can reduce coastal flood risks. Estuar. Coast. Shelf Sci. 242, 106844 (2020)
- Chow, J. Mangrove management for climate change adaptation and sustainable development in coastal zones. J. Sustain. For. 37, 139–156 (2018).
- Dasgupta, S., Islam, M. S., Huq, M., Huque Khan, Z. & Hasib, M. R. Quantifying the protective capacity of mangroves from storm surges in coastal Bangladesh. PLoS One 14, e0214079 (2019).
Social Risks of Coastal Vegetated Ecosystems
Efforts around restoring natural ecosystems are often met with social acceptance and public support, especially when compared to other climate solutions seen as “tampering with nature”1. This may be in part due to the minimal risks (real and perceived) associated with restoring natural ecosystems. While some risks, outlined below, do exist, there may be a higher tolerance for such risks given the long list of potential benefits and co-benefits (both environmentally and socially).
- Potential for conflict with other industries (aquaculture, fishing, tourism etc.); However, conflicts may be mitigated with participatory approaches to planning and implementation.
- Potential for inequity in benefits: Macreadie et al. (2022) points to concerns around the distribution of benefits from any given blue carbon project and whether benefits are evenly distributed or whether activities reinforce or add to social inequity2. Similarly, there may be confusion around who “owns” the blue carbon and who has rights to control transactions of credits resulting from blue carbon projects. This may be particularly challenging in marine spaces where the movement of carbon must be considered.
- Wolske, K. S., K. T. Raimi, V. Campbell-Arvai, and P. S. Hart. 2019. “Public support for carbon dioxide removal strategies: the role of tampering with nature perceptions.” Climatic Change 152 (3-4):345-361. doi:10.1007/s10584-019-02375-z.
- Macreadie, Peter I., et al. “Operationalizing Marketable Blue Carbon.” One Earth, vol. 5, no. 5, 2022, pp. 485–492, https://doi.org/10.1016/j.oneear.2022.04.005.
First-Order Priorities Common to All Coastal Vegetated Ecosystems
- Develop accessible (economically and geographically) sensors and technologies to measure greenhouse gas exchanges and fluxes both temporally and spatially at high enough resolution to create predictive models for net carbon sequestration and durability.
- Create models that account for future impacts/effects of climate change and land-use change (e.g., coastal squeeze)
- Create new frameworks (e.g., seascape1 or watershed approaches) both for research and financial mechanisms that account for intersystem linkages and take a more holistic approach to measuring carbon associated with coastal vegetated ecosystems.
- Currently, ecosystems are considered and studied individually which can cause issues when thinking about the movement of carbon between systems
- Investigate and characterize the historical or pre-industrial ecosystem locations and ranges (which in many cases are currently unknown) in order to establish baselines for restoration projects.
- In some instances, these prior states may need to be derived from historical sources including more traditional data sets and non-traditional data sets like paintings2.
- Huxham, M., et al. “Carbon in the coastal seascape: How interactions between mangrove forests, seagrass meadows and tidal marshes influence carbon storage.” Current Forestry Reports, vol. 4, no. 2, 2018, pp. 101–110, https://doi.org/10.1007/s40725-018-0077-4.
- Duarte, C. M. et al. Rebuilding marine life. Nature 580, 39–51 (2020)
Current Financing Landscape & Common Challenges
Current Landscape
- Verra: In 2020 Verra, a standard for certifying carbon credits, released its first blue carbon conservation methodology, an update to the VCS REDD+ Methodology Framework. Verra has also formed a blue carbon Working Group (2020) that explores opportunities for coastal wetland restoration and conservation as well as developed the Wetland Restoration and Conservation Requirements. Addressing blue carbon in the open ocean, Verra created an Ocean Carbon Working Group as well as the Seascape Carbon Initiative (co-led by Verra, Silvestrum Climate Associates, Blue Marine Foundation, the blue carbon Initiative, and Oceans 2050)4.
- Gold Standard: Gold Standard is a carbon offset standard for non-governmental emission reductions projects. Gold Standard has an approved methodology for the certification of mangrove projects and are exploring other blue carbon opportunities.
- Plan Vivo: Plan Vivo is a certification body that certifies projects against the Plan Vivo Standard, a framework for community and smallholder land-use and forestry projects. In 2014, Plan Vivo certified the world’s first blue carbon project, Mikoko Pamoja (Kenya), for mangrove conservation5.
- Fair Carbon: Fair Carbon supports the development of blue Carbon projects by increasing the accessibility of information and transparency6. This includes their Global Blue Carbon Projects map which allows access to all publicly registered blue carbon projects across multiple voluntary carbon market registries. They also have a number of other resources around carbon credits for blue carbon.
- Sustainable Development Mechanism (formerly the Clean Development Mechanism): This is a compliance market designed for shorter-term emissions credits.
- Nationally Determined Contributions (NDCs): These are the climate actions detailed and executed by countries participating in the Paris Agreement and serve as the primary mechanism for meeting the goals set out in the Paris Agreement. The restoration of blue carbon ecosystems may help mitigate and adapt to climate change, however their integration into Nationally Determined Contributions remains underdeveloped7. A 2022 report from thirty three experts highlights the need for “genuine participation by communities, inclusive project governance, integration of local work into national policies and practices, sustaining livelihoods and income (for example through the voluntary carbon market and/or national Payment for Ecosystem Services and other types of financial compensation systems) and simplification of carbon accounting and verification methodologies to lower barriers to entry8” in order for blue carbon projects to be responsibly and successfully integrated into Nationally Determined Contributions.
- New efforts to improve the quality of carbon credits and the voluntary carbon market are being discussed and developed9. The integrity of each carbon credit will be critical to enable the carbon market to address climate change. New guidance has been released in 2023 to ensure carbon credits deliver on their claims. The Integrity Council for the Voluntary Carbon Market released its Core Carbon Principles10 that set a global threshold to define what a high-integrity carbon credit is.
Challenges
- Verification of the amount of carbon sequestered and its durability can be extremely challenging across coastal vegetated ecosystems but is necessary for effective (in terms of mitigating climate change) carbon markets.
- Ensuring fairness and equity in benefits distribution
- https://www.wetlands-initiative.org/nutrient-credit-trading
- https://www.calandtrusts.org/conservation-basics/conservation-tools/mitigationbanks/
- https://www.weforum.org/press/2023/03/new-initiative-to-help-government-and-business-navigate-untapped-blue-carbon-and-restore-coastal-ecosystems/
- https://verra.org/programs/verified-carbon-standard/area-of-focus-blue-carbon/
- https://www.planvivo.org/news/our-statement-on-blue-carbon
- https://faircarbon.org/
- Dencer-Brown, Amrit Melissa, et al. “Integrating Blue: How Do We Make Nationally Determined Contributions Work for Both Blue Carbon and Local Coastal Communities?” Ambio, vol. 51, no. 9, 2022, pp. 1978–1993, https://doi.org/10.1007/s13280-022-01723-1.
- Dencer-Brown, Amrit Melissa, et al. “Integrating Blue: How Do We Make Nationally Determined Contributions Work for Both Blue Carbon and Local Coastal Communities?” Ambio, vol. 51, no. 9, 2022, pp. 1978–1993, https://doi.org/10.1007/s13280-022-01723-1.
- https://blogs.edf.org/climate411/2023/08/01/navigating-the-core-carbon-principles-and-the-landscape-of-guidance-toward-a-high-integrity-carbon-market/
- https://icvcm.org/the-core-carbon-principles/
Seaweed: Natural Seaweed Stands & Coastal Seaweed Farms
Overview
Seaweeds use photosynthesis to convert dissolved carbon in the surrounding seawater into organic compounds and living tissue1. As dissolved carbon is removed from the ocean, it is replaced by carbon dioxide from the atmosphere. It is through this process that seaweed can remove CO2 from the atmosphere. The ultimate fate of carbon sequestered in living seaweed is varied and includes becoming remineralized, or converted back, to CO2 via grazing, exuded as dissolved organic carbon, and being transported to the deep sea as particulate matter. The rate and proportion of carbon through each of these pathways remains uncertain2, though a 2016 study from Krause-Jensen and Duarte sheds some light on possible export efficiencies3. What’s more, the rate and proportion of carbon that goes through each of the pathways mentioned are not only uncertain but context-dependent. For an in-depth exploration of the readiness of seaweed as a blue carbon solution (both natural stands and farmed) see Fujita et al. 20234.
Note that this road map will exclusively look at the ability of natural seaweed stands and coastal seaweed farms to passively sequester CO2 (without active human intervention). For an in-depth look at growing seaweed at large scale to be sequestered using active human intervention (such as deep sea sinking and production of algal biochar), please see the Macroalgae Cultivation and Carbon Sequestration Road Map.
- Paine, E. R., Schmid, M., Boyd, P. W., Diaz-Pulido, G., & Hurd, C. L. (2021). Rate and fate of dissolved organic carbon release by seaweeds: A missing link in the coastal ocean carbon cycle. Journal of Phycology, 57(5), 1375–1391. doi.org/10.1111/ jpy.13198
- Macreadie, P. I., Anton, A., Raven, J. A., Beaumont, N., Connolly, R. M., Friess, D. A., Kelleway, J. J. et al. 2019. The future of blue carbon science. Nat. Commun. 10:3998.
- Krause-Jensen, D., & Duarte, C. M. (2016). Substantial role of macroalgae in marine carbon sequestration. Nature Geoscience, 9. nature.com/articles/ngeo2790
- Fujita, Rod, et al. “Seaweed Blue Carbon: Ready? Or Not?” Marine Policy, vol. 155, 2023, p. 105747, https://doi.org/10.1016/j.marpol.2023.105747.
Natural Seaweed Stands
Here, natural seaweed stands denotes seaweed naturally occurring in the ocean, also commonly referred to as kelp forests.
Mechanisms for CDR
- Reforestation of natural seaweed stands is the process of assisting in the recovery of seaweed stands that have been degraded, damaged, or destroyed, and is a type of restoration.
- The reforestation of natural seaweed stands to increase carbon storage involves many context-specific factors that may complicate such efforts. Factors that will affect the growth and ultimate success of seaweed include climate change, disease, grazers, and predator interactions, etc.1. It is also highly species dependent with some species of seaweed better suited than others for CDR. This complex assemblage of factors, unique across geographies, will require restoration efforts to be highly tailored.
- A 2022 meta-analysis of seaweed restoration projects found a high success rate of restoration projects, however many of the reported projects were small in scale and these findings may not reflect failed restoration projects that were not reported2.
- The Nature Conservancy’s guidance on developing kelp restoration projects3
- One emerging method for restoring degraded seaweed stands is seeding small rocks with juvenile kelp, often termed ‘Green Gravel’. See work by the Green Gravel Action Group.
- Another proposed technique actively removes urchins from seaweed stands to allow for the settlement of juvenile seaweed and subsequent regrowth. See Urchinomics for one approach.
- Increasing productivity of existing seaweed stands via genetic manipulation
- There is current research on improving strain selection for enhanced carbon sequestration, productivity, or resiliency of seaweed. See ongoing research by Charles Yarish and lab at the University of Connecticut.
CDR Potential
Estimated Sequestration Potential: 0 - 0.018 Gt CO2e /year4
Sequestration Durability: Potentially hundreds of years, however, more studies are needed to understand how much seaweed becomes “refractory”, or resistant to degradation on the timescale of hundreds of years5.
Environmental Co-Benefits
Restoration of natural seaweed stands may generate many benefits for surrounding marine ecosystems. While we are calling these “co-benefits” here, it should be noted that some of these benefits may be so significant that these may in fact be the main benefits of restoration. (Note that there are significantly more co-benefits in blue carbon than any of the other marine CDR Road Maps.)
- Potential amelioration of ocean acidification6
- Contribution to biodiversity7
- Contribution to habitat provisioning8
- Contribution to heavy metal and nutrient pollution removal9,10
- Contribution to fishery enhancement11,12
- Improved water clarity through the facilitation of settlement of fine sediments13
- Nutrient cycling14
- Reducing biodiversity loss15
- Restoring the role of marine organisms in the carbon cycle16
Environmental Risks
In contrast to other proposed marine CDR pathways, there are not as many apparent environmental risks to reforesting natural seaweed stands for carbon dioxide removal. However, proper restoration techniques which take context-specific factors into consideration are critical to ensuring no or limited negative environmental impacts. As with any CDR pathway, incremental scale-up with careful monitoring and verification of impacts will be critical to mitigate risks. Possible environmental risks may include:
- Species entanglement
- Nutrient competition
- Smothering of adjacent sensitive ecosystems
- Negative effects of organic exudates on neighboring ecosystems
- If hard bottom substrates are needed (for seaweed to attach to) this could disturb soft bottom habitats. See examples of artificial reef creation to mitigate the loss of natural reef and restore seaweed.
Social Co-Benefits
Reforestation of natural seaweed stands may generate benefits for surrounding communities and economies. While we are calling these “co-benefits” here, it should be noted that some of these benefits may be so significant (while carbon sequestration feasibility and efficacy remain unknown) that these may in fact be the main benefits of restoration. Many of the environmental benefits relate directly to social benefits, such as fisheries enhancement and coastal protection. (Note that there are significantly more co-benefits in blue carbon than any of the other CDR Road Maps.)
- Increased adaptive capacity for communities to handle climate change and natural disasters via mechanisms such as wave attenuation for coastal protection17, providing a refugia from acidified waters that benefits shellfish18, mitigation of dead zones by production of dissolved oxygen19, or possibly providing shading from seaweed blades to create a thermal refugia during marine heat waves20
- Job creation and economic stimulation from enhanced fisheries and tourism (of particular value to coastal communities that are dependent on marine-based livelihoods)
- Cultural / intrinsic / recreational value
- Education and research – the pursuit of restoring blue carbon for CDR will naturally add value to the existing body of research and can provide valuable educational experiences for the upcoming generation of scientists and conservationists.
Social Risks
Efforts around restoring natural ecosystems are often met with social acceptance and public support, especially when compared to other climate solutions seen as “tampering with nature”21. This may be in part due to the minimal risks (real and perceived) associated with restoring natural ecosystems. While some risks, outlined below, do exist, there may be a higher tolerance for such risks given the long list of potential benefits and co-benefits (both environmentally and socially).
- Potential for inequity in benefits: Macreadie et al. (2022) points to concerns around the distribution of benefits from any given blue carbon project and whether benefits are evenly distributed or whether activities reinforce or add to social inequity22. Similarly, there may be confusion around who “owns” the blue carbon and who has rights to control transactions of credits resulting from blue carbon projects. This may be particularly challenging in marine spaces where the movement of carbon must be taken into account.
- Potential for conflict with other uses such as commercial fisheries, shipping, marine renewable energy, and mining23 however, there may be synergies for some industries, like utilizing infrastructure from nearby renewable energy for monitoring.
Key Knowledge Gaps
Adapted from EDF 2022 Carbon Sequestered by Seaweed24
- What is the best way to measure rates of carbon sequestration by natural seaweed stands?
- Which of the carbon fluxes between natural seaweed stands and their environment are well quantified, and which are not?
- How does carbon sequestered by natural seaweed stands compare with other ocean-based carbon sequestration pathways?
- Is the restoration of natural seaweed stands a durable and feasible enough pathway to help stabilize the climate?
*Note that all of these are highly dependent upon what species of seaweed is being considered.
First-Order Priorities
*Adapted from EDF 2022 Carbon Sequestered by Seaweed24
- Conduct research to better understand and characterize carbon fluxes in natural seaweed stands
- Determine the best way to measure carbon sequestration rates by natural seaweed stands*
- Determine which of the carbon fluxes relevant to carbon sequestration by seaweeds are well quantified and which are not. This includes carbon fluxes from the atmosphere to ocean, ocean to seaweed, seaweed to microbial and other food webs, and seaweed to deep water*.
- Characterize how the potential carbon sequestration by natural seaweed stands compares with other ocean-based carbon sequestration pathways*
- Determine the durability of carbon sequestered via natural seaweed stands and if it is long enough to help stabilize the climate*
- Accelerate the innovation and testing of new technology that can aid in restoration projects
- Conduct further field testing of techniques such as green gravel for afforestation of kelp forests.
- Conduct more research on the efficacy of utilizing genetic manipulation and biotechnology to enhance seaweed carbon capture efficiencies.
- Fujita, Rod, et al. “Seaweed Blue Carbon: Ready? Or Not?” Marine Policy, vol. 155, 2023, p. 105747, https://doi.org/10.1016/j.marpol.2023.105747.
- A.M. Eger, E.M. Marzinelli, C. Hartvig, C. Fagerli, D. Fujita, Global kelp forest restoration: past lessons, present status, and future directions, in: Biological Reviews, 97, 2022, pp. 1449–1475, https://doi.org/10.1111/brv.12850.
- Gleason, M., Caselle, J.E., Heady, W.N., Saccomanno, V.R., Zimmerman, J., Anoush McHugh, T. (2021). A Structured Approach for Kelp Restoration and Management Decisions in California. Nature Conservancy and UCSB Marine Science Institute. https://www.scienceforconservation.org/products/structured-decisionmaking-kelp
- Hoegh-Guldberg, O., Northrop, E. et al. 2023. "The ocean as a solution to climate change: Updated opportunities for action." Special Report. Washington, DC: World Resources Institute. Available online at https://oceanpanel.org/publication/ocean-solutions-to-climate-change
- Fujita, R. M., Collins, J. R., Kleisner, K. M., Rader, D. N., Augyte, S., and Brittingham, P. A., 2022. Carbon sequestration by seaweed: background paper for the Bezos Earth Fund - EDF workshop on seaweed carbon sequestration. Environmental Defense Fund, New York, NY. URL
- Mongin, M., Baird, M. E., Hadley, S., & Lenton, A. (2016). Optimising reef-scale CO2 removal by seaweed to buffer ocean acidification. IOPscience. iopscience.iop.org/ article/10.1088/1748-9326/11/3/034023
- Theuerkauf, S. J., Barrett, L. T., Alleway, H. K., Costa-Pierce, B. A., St. Gelais, A., & Jones, R. C. (2021). Habitat value of bivalve shellfish and seaweed aquaculture for fish and invertebrates: Pathways, synthesis and next steps. Reviews in Aquaculture. onlinelibrary.wiley.com/doi/10.1111/raq.12584
- Langton, R., Augyte, S., Price, N., Forster, J., Noji, T., & Grebe, G. (2019). An Ecosystem Approach to the Culture of Seaweed. NOAA, 30.
- Zheng, Y., Jin, R., Zhang, X., & Wang, Q. (2019). The considerable environmental benefits of seaweed aquaculture in China. Stochastic Environmental Research and Risk Assessment, 33. doi.org/10.1007/s00477-019-01685-z
- Jiang, Z., Liu, J., Li, S., Chen, Y., Du, P., Zhu, Y., Liao, Y., Chen, Q., Shou, L., Yan, X., Zeng, J., & Chen, J. (2020). Kelp cultivation effectively improves water quality and regulates phytoplankton community in a turbid, highly eutrophic bay. The Science of the Total Environment, 707, 135561. doi. org/10.1016/j.scitotenv.2019.135561
- Rimmer MA, Larson S, Lapong I, Purnomo AH, Pong-Masak PR, Swanepoel L, Paul NA. Seaweed Aquaculture in Indonesia Contributes to Social and Economic Aspects of Livelihoods and Community Wellbeing. Sustainability. 2021; 13(19):10946. https://doi.org/10.3390/su131910946
- Theuerkauf, Seth J., et al. “Habitat value of bivalve shellfish and seaweed aquaculture for fish and invertebrates: Pathways, synthesis and next steps.” Reviews in Aquaculture, vol. 14, no. 1, 2021, pp. 54–72, https://doi.org/10.1111/raq.12584.
- Jiang, Z., Liu, J., Li, S., Chen, Y., Du, P., Zhu, Y., Liao, Y., Chen, Q., Shou, L., Yan, X., Zeng, J., & Chen, J. (2020). Kelp cultivation effectively improves water quality and regulates phytoplankton community in a turbid, highly eutrophic bay. The Science of the Total Environment, 707, 135561. doi. org/10.1016/j.scitotenv.2019.135561
- Cotas, João, et al. “Ecosystem Services provided by seaweeds.” Hydrobiology, vol. 2, no. 1, 2023, pp. 75–96, https://doi.org/10.3390/hydrobiology2010006.
- National Academies of Sciences, Engineering, and Medicine 2022. A Research Strategy for Ocean-based Carbon Dioxide Removal and Sequestration. Washington, DC: The National Academies Press. https://doi.org/10.17226/26278.
- National Academies of Sciences, Engineering, and Medicine 2022. A Research Strategy for Ocean-based Carbon Dioxide Removal and Sequestration. Washington, DC: The National Academies Press. https://doi.org/10.17226/26278.
- Denny, M. Wave-Energy Dissipation: Seaweeds and Marine Plants Are Ecosystem Engineers. Fluids 2021, 6, 151. https://doi.org/10.3390/fluids6040151
- Edworthy, Carla, et al. “The role of macroalgal habitats as ocean acidification refugia within coastal seascapes.” Cambridge Prisms: Coastal Futures, vol. 1, 2023, https://doi.org/10.1017/cft.2023.9.
- Predicted/possible impact
- Predicted/possible impact
- Wolske, K. S., K. T. Raimi, V. Campbell-Arvai, and P. S. Hart. 2019. “Public support for carbon dioxide removal strategies: the role of tampering with nature perceptions.” Climatic Change 152 (3-4):345-361. doi:10.1007/s10584-019-02375-z.
- Macreadie, Peter I., et al. “Operationalizing Marketable Blue Carbon.” One Earth, vol. 5, no. 5, 2022, pp. 485–492, https://doi.org/10.1016/j.oneear.2022.04.005.
- National Academies of Sciences, Engineering, and Medicine 2022. A Research Strategy for Ocean-based Carbon Dioxide Removal and Sequestration. Washington, DC: The National Academies Press. https://doi.org/10.17226/26278.
- Fujita, R. M., Collins, J. R., Kleisner, K. M., Rader, D. N., Mejaes, A., Augyte, S., and Brittingham, P. A., 2022. Carbon sequestration by seaweed: background paper for the Bezos Earth Fund - EDF workshop on seaweed carbon sequestration. Environmental Defense Fund, New York, NY. https://www.edf.org/sites/default/files/2022-10/Carbon%20Sequestration%20by%20Seaweed.pdf
Coastal Seaweed Farms
Mechanism for CDR
Expansion of seaweed farming
- Possibly the most promising pathway to increase carbon sequestration by seaweed due to rapid advances in infrastructure, farm operations, and monitoring.
- Achieving additional, passive, carbon sequestration would require biomass to be left in the ocean long enough for fragmentation, transport, and burial to occur.
CDR Potential
Estimated Sequestration Potential: 0.00144 – 0.0079 Gt CO2e/year1
Sequestration Durability: Potentially hundreds of years, however, more studies are needed to understand how much seaweed becomes “refractory”, or resistant to degradation on the timescale of hundreds of years2.
Environmental Co-Benefits
The creation or expansion of seaweed farms may generate benefits for surrounding marine ecosystems, including but not limited to:
- Potential amelioration of ocean acidification3
- Contribution to biodiversity4
- Contribution to habitat provisioning5
- Contribution to heavy metal and nutrient pollution removal6,7
- Contribution to fishery enhancement8,9
- Improved water clarity through the facilitation of settlement of fine sediments10
- Nutrient cycling11
- Restoring the role of marine organisms in the carbon cycle12
Environmental Risks
- Burning of fossil fuels associated with production activities (harvest, transport, processing)
- Production of CH4, N2O, and other potentially hazardous gases by the macroalgae
- Impacts to biodiversity and ecosystem function from farming operations may include: enhanced disease and parasite risk, alteration of population genetics, introduction of non-native species into new environments
- Enhancement in epiphytic calcifiers that could offset carbon sequestration through calcification-induced CO2 release.
- Reduced phytoplankton production in and around seaweed farms due to competition for nutrients and light
- Changes in light and nutrient availability (including possible changes in ocean albedo due to canopy cover)
- Entanglement of marine megafauna
- Addition of noise pollution due to vessel traffic and machinery
Social Co-Benefits
Seaweed farming may generate many benefits for surrounding communities and economies.
- Basis for circular marine bio-economies, generating multiple benefits13 including integrated multi-trophic farming efforts to collocate multiple species (i.e., seaweed and shellfish)
- Education and research – the pursuit of restoring blue carbon for CDR will naturally add value to the existing body of research and can provide valuable educational experiences for the upcoming generation of scientists and conservationists.
Social Risks
While some risks, outlined below, do exist, there may be a higher tolerance for such risks given the long list of potential benefits and co-benefits (both environmentally and socially).
- Potential for conflict with other industries (fishing, tourism etc.)14
- Potential for conflict with other uses such as commercial fisheries, shipping, marine renewable energy, and mining15 (However, note there are also possible synergies that may exist such as co-location of seaweed farms with compatible marine infrastructure types such as the moorings for wind energy, which could reduce costs for both industries.)
Challenges
- Scaling
- Rough seas, light and nutrient availability, and the availability of space are all constraints.
- Measuring Carbon
- Difficulties in measuring and verifying how much carbon is being sequestered passively by the presence of seaweed farms.
Key Knowledge Gaps
*Adapted from EDF 2022 Carbon Sequestered by Seaweed16
- What is the best way to measure rates of carbon sequestration by seaweed farms?
- Which of the carbon fluxes between seaweed farms and their environment are well quantified and which are not?
- How does carbon sequestered by seaweed farms compare with other ocean-based carbon sequestration pathways?
First-Order Priorities
*Adapted from EDF 2022 Carbon Sequestered by Seaweed16
- Investigate and characterize carbon fluxes in coastal seaweed farms
- Determine the best ways to measure rates of carbon sequestered by seaweed farms*
- Investigate which carbon flows relevant to quantifying carbon sequestration by seaweed farms are well documented and which remain uncertain*
- Estimate total greenhouse gas emissions (including N2O and CH4) and carbon sequestration associated with seaweed farms17*
- Hoegh-Guldberg, O., Northrop, E. et al. 2023. "The ocean as a solution to climate change: Updated opportunities for action." Special Report. Washington, DC: World Resources Institute. Available online at https://oceanpanel.org/publication/ocean-solutions-to-climate-change
- Fujita, R. M., Collins, J. R., Kleisner, K. M., Rader, D. N., Augyte, S., and Brittingham, P. A., 2022. Carbon sequestration by seaweed: background paper for the Bezos Earth Fund - EDF workshop on seaweed carbon sequestration. Environmental Defense Fund, New York, NY. URL
- Mongin, M., Baird, M. E., Hadley, S., & Lenton, A. (2016). Optimising reef-scale CO2 removal by seaweed to buffer ocean acidification. IOPscience. iopscience.iop.org/ article/10.1088/1748-9326/11/3/034023
- Theuerkauf, S. J., Barrett, L. T., Alleway, H. K., Costa-Pierce, B. A., St. Gelais, A., & Jones, R. C. (2021). Habitat value of bivalve shellfish and seaweed aquaculture for fish and invertebrates: Pathways, synthesis and next steps. Reviews in Aquaculture. onlinelibrary.wiley.com/doi/10.1111/raq.12584
- Langton, R., Augyte, S., Price, N., Forster, J., Noji, T., & Grebe, G. (2019). An Ecosystem Approach to the Culture of Seaweed. NOAA, 30.
- Zheng, Y., Jin, R., Zhang, X., & Wang, Q. (2019). The considerable environmental benefits of seaweed aquaculture in China. Stochastic Environmental Research and Risk Assessment, 33. doi.org/10.1007/s00477-019-01685-z
- Jiang, Z., Liu, J., Li, S., Chen, Y., Du, P., Zhu, Y., Liao, Y., Chen, Q., Shou, L., Yan, X., Zeng, J., & Chen, J. (2020). Kelp cultivation effectively improves water quality and regulates phytoplankton community in a turbid, highly eutrophic bay. The Science of the Total Environment, 707, 135561. doi. org/10.1016/j.scitotenv.2019.135561
- Rimmer MA, Larson S, Lapong I, Purnomo AH, Pong-Masak PR, Swanepoel L, Paul NA. Seaweed Aquaculture in Indonesia Contributes to Social and Economic Aspects of Livelihoods and Community Wellbeing. Sustainability. 2021; 13(19):10946. https://doi.org/10.3390/su131910946
- Theuerkauf, Seth J., et al. “Habitat value of bivalve shellfish and seaweed aquaculture for fish and invertebrates: Pathways, synthesis and next steps.” Reviews in Aquaculture, vol. 14, no. 1, 2021, pp. 54–72, https://doi.org/10.1111/raq.12584.
- Jiang, Z., Liu, J., Li, S., Chen, Y., Du, P., Zhu, Y., Liao, Y., Chen, Q., Shou, L., Yan, X., Zeng, J., & Chen, J. (2020). Kelp cultivation effectively improves water quality and regulates phytoplankton community in a turbid, highly eutrophic bay. The Science of the Total Environment, 707, 135561. doi. org/10.1016/j.scitotenv.2019.135561
- Cotas, João, et al. “Ecosystem Services provided by seaweeds.” Hydrobiology, vol. 2, no. 1, 2023, pp. 75–96, https://doi.org/10.3390/hydrobiology2010006.
- National Academies of Sciences, Engineering, and Medicine 2022. A Research Strategy for Ocean-based Carbon Dioxide Removal and Sequestration. Washington, DC: The National Academies Press. https://doi.org/10.17226/26278.
- Zhang, Xueqian, and Marianne Thomsen. 2019. "Biomolecular Composition and Revenue Explained by Interactions between Extrinsic Factors and Endogenous Rhythms of Saccharina latissima" Marine Drugs 17, no. 2: 107. https://doi.org/10.3390/md17020107
- National Academies of Sciences, Engineering, and Medicine 2022. A Research Strategy for Ocean-based Carbon Dioxide Removal and Sequestration. Washington, DC: The National Academies Press. https://doi.org/10.17226/26278.
- National Academies of Sciences, Engineering, and Medicine 2022. A Research Strategy for Ocean-based Carbon Dioxide Removal and Sequestration. Washington, DC: The National Academies Press. https://doi.org/10.17226/26278.
- Fujita, R. M., Collins, J. R., Kleisner, K. M., Rader, D. N., Mejaes, A., Augyte, S., and Brittingham, P. A., 2022. Carbon sequestration by seaweed: background paper for the Bezos Earth Fund - EDF workshop on seaweed carbon sequestration. Environmental Defense Fund, New York, NY. https://www.edf.org/sites/default/files/2022-10/Carbon%20Sequestration%20by%20Seaweed.pdf
- Duarte, Carlos M., et al. Carbon Burial in Sediments below Seaweed Farms, 2023, https://doi.org/10.1101/2023.01.02.522332.
Animal Carbon: Whales & Fishes
Overview
Whales
Whales are thought to play a role in the carbon cycle both directly (living biomass and whale falls) and indirectly (the whale pump, the whale conveyor belt, and bioturbation), however the extent of this role remains uncertain1. A 2022 report from The Environmental Defense Fund2 found there to be fewer than 10 scientific studies from which to draw any broad conclusions in this area and cautioned against the creation of a carbon credit system based on whale carbon sequestration. A 2020 study estimates that restoring baleen whale populations to pre-whaling abundances could sequester 0.032 Gt CO2/year, including carbon sequestered from fertilization via whale feces3. However, rebuilding whale populations is sure to elicit many other environmental and social benefits, explored further on in this road map.
Mechanisms for CDR
Direct Sequestration
- Living Biomass: Due to their size and longevity, whales can store large amounts of carbon for long periods of time in their bodies as biomass. While whaling indeed resulted in a loss of carbon stored in whales, there is not clear evidence to suggest that whaling was a net source of CO2 to the atmosphere4.
- Whale Falls: Whale falls, wherein whale carcasses sink to the ocean floor and their carbon-rich biomass is reallocated in various ways, is the “most scientifically comprehensible” of the pathways (direct vs indirect sequestration)5 with a 2010 study estimating that restoration of large baleen whale populations to pre-whaling numbers could sequester 0.0006 Gt CO2e /year.
Indirect Sequestration
- Whale Pump: This is the term often used to describe the role that whales play in cycling nutrients through the ocean via their feces. The whale pump hypothesis sits on two assumptions that have not yet been verified through empirical observations6. The first assumption is around the efficacy of surface water fertilization and subsequent primary production via whale feces. The second assumption concerns the fate of carbon export from primary production.
- WhaleX Ocean Nourishment synthesizes limiting nutrients (nitrogen, phosphorous, iron, and silica) and disperses them across nutrient poor regions of the ocean, simulating the dispersion of nutrients via whale feces.
- Whale Conveyor Belt: The whale conveyor belt is based on the idea that as whales migrate yearly, they move nutrients around the ocean, potentially increasing iron availability for phytoplankton growth7.
- Bioturbation: Bioturbation is the process through which whale feeding behaviors stimulate the seabed and resuspend sediments8. Conceptually, this is similar to the whale pump, but the pool of nutrients comes from seafloor sediments instead of whale feces9,10.
Rebuilding Whale Populations: Increasing whale populations would likely amplify their role in the carbon cycle, including any sequestration.
- Increasing Krill Abundance:
- A 2022 article by Savoca11 suggests krill as a limiting factor to whale population growth. Savoca points to studies that suggest a close relationship between whales and krill wherein whales provide necessary nutrients for krill to thrive. This is further complicated by competition between krill fisheries and whales in the Southern Ocean.
Reducing Ship Strike: A 2001 study found that in some areas, more than one third of all fin whale and right whale strandings involve ship strike. This can especially impact small populations of whales such as the northern right whale in the western North Atlantic12. Measures that require large, motorized vessels to slow their speeds in particularly vulnerable areas may be beneficial to whale populations.
CDR Potential
Estimated Sequestration Potential: 0.032 Gt CO2e /year, including carbon sequestered from fertilization13
Sequestration Durability: 10 – 100 years14
Challenges
- Difficult to find and track outcomes from whale fertilization events (e.g., feces)
- Tracking the fate of carbon through the water column
Key Knowledge Gaps
Adapted from Pearson et al. 2022
- How effective are whales at creating and maintaining primary production hotspots that lead to enhanced carbon sequestration?
- How bioavailable are whale-derived nutrients?
- What is the carbon flux from cetaceans to the atmosphere?
First-Order Priorities
*Adapted from Pearson et al. 2022
- Investigate primary production hot spots from whales via determination of fecal nutrients to carbon recycling versus export*
- Conduct additional field and laboratory studies to ascertain the bioavailability of krill-derived iron in whale feces*
- Measure the carbon flux from cetaceans to the atmosphere via respiration to understand cetaceans’ net capacity for CO2 removal*
- Meynecke J-O, Samanta S, de Bie J, Seyboth E, Prakash Dey S, Fearon G, Vichi M, Findlay K, Roychoudhury A and Mackey B (2023) Do whales really increase the oceanic removal of atmospheric carbon? Front. Mar. Sci. 10:1117409. doi: 10.3389/fmars.2023.1117409
- Collins, J. R., Boenish, R. E., Fujita, R. M., Rader, D. N., and Moore, L. A. 2022. Natural climate solutions in the open ocean: scientific knowledge and opportunities surrounding four potential pathways for carbon dioxide removal or avoided emissions. Environmental Defense Fund, New York, NY. https://www.edf.org/sites/default/files/2022-10/Natural%20Climate%20Solutions%20in%20the%20Open%20Ocean.pdf
- Durfort, Anaelle, et al. The Collapse and Recovery Potential of Carbon Sequestration by Baleen Whales in the Southern Ocean, 2020, https://doi.org/10.21203/rs.3.rs-92037/v1.
- Pearson, Heidi C., et al. “Whales in the Carbon Cycle: Can Recovery Remove Carbon Dioxide?” Trends in Ecology & Evolution, vol. 38, no. 3, 2023, pp. 238–249, https://doi.org/10.1016/j.tree.2022.10.012.
- Collins, J. R., Boenish, R. E., Fujita, R. M., Rader, D. N., and Moore, L. A. 2022. Natural climate solutions in the open ocean: scientific knowledge and opportunities surrounding four potential pathways for carbon dioxide removal or avoided emissions. Environmental Defense Fund, New York, NY. https://www.edf.org/sites/default/files/2022-10/Natural%20Climate%20Solutions%20in%20the%20Open%20Ocean.pdf
- Collins, J. R., Boenish, R. E., Fujita, R. M., Rader, D. N., and Moore, L. A. 2022. Natural climate solutions in the open ocean: scientific knowledge and opportunities surrounding four potential pathways for carbon dioxide removal or avoided emissions. Environmental Defense Fund, New York, NY. https://www.edf.org/sites/default/files/2022-10/Natural%20Climate%20Solutions%20in%20the%20Open%20Ocean.pdf
- Roman J, Estes JA, Morissette L, Smith C, Costa D, McCarthy J, Nation JB, Nicol S, Pershing A, Smetacek V. Whales as marine ecosystem engineers. Frontiers in Ecology and the Environment. 2014 Sep;12(7):377-85.
- Meynecke J-O, Samanta S, de Bie J, Seyboth E, Prakash Dey S, Fearon G, Vichi M, Findlay K, Roychoudhury A and Mackey B (2023) Do whales really increase the oceanic removal of atmospheric carbon? Front. Mar. Sci. 10:1117409. doi: 10.3389/fmars.2023.1117409
- Nelson, C. H., and Johnson, K. R. (1987). Whales and walruses as tillers of the sea floor. Sci. Am. 256, 112–117. doi: 10.1038/scientificamerican0287-112
- Alter, S. E., Rynes, E., and Palumbi, S. R. (2007). DNA Evidence for historic population size and past ecosystem impacts of gray whales. Proc. Natl. Acad. Sci. 104, 15162–15167. doi: 10.1073/pnas.0706056104
- Savoca, Matthew. 2022. “The fall of the great ocean farmers.” The Marine Biologist. ISSN: 2052-5273.
- Laist, David W., et al. “Collisions between Ships and Whales.” Marine Mammal Science, vol. 17, no. 1, 2001, pp. 35–75, https://doi.org/10.1111/j.1748-7692.2001.tb00980.x.
- Durfort, A., G. Mariani, M. Troussellier, V. Tulloch, and D. Mouillot. 2021. “The Collapse and Recovery Potential of Carbon Sequestration by Baleen Whales in the Southern Ocean.” Preprint. doi.org/10.21203/rs.3.rs-92037/v1.
- Cross, J.N., Sweeney, C., Jewett, E.B., Feely, R.A., McElhany, P., Carter, B., Stein, T., Kitch, G.D., and Gledhill, D.K., 2023. Strategy for NOAA Carbon Dioxide Removal Research: A white paper documenting a potential NOAA CDR Science Strategy as an element of NOAA’s Climate Interventions Portfolio. NOAA Special Report. NOAA, Washington DC. DOI: 10.25923/gzke-8730
Fishes
While fishes have not traditionally been considered as important parts of the ocean carbon cycle / biological pump1, there are several recent studies, as highlighted in the 2022 Environmental Defense Fund report “Natural Climate Solutions in the Open Ocean”, that focus on the geochemical contributions of fishes to the global carbon cycle23456. Many of the mechanisms by which fish are thought to contribute to carbon cycling are similar to whales (carbon stored in living biomass, bioturbation, carcasses falling to ocean floor, fertilization through feces) but also include contribution by intestinal precipitation of calcium carbonates7.
There is still uncertainty around the specifics of mesopelagic fish-mediated carbon export, however the 2022 Environmental Defense Fund report suggests there is enough evidence of these species’ large contributions to global sequestration to warrant “the immediate pursuit of limitations or prohibitions on their harvest”.
Mechanism for CDR
Limitations or prohibitions on harvest may increase the living biomass of fish, inducing higher natural long-term carbon sequestration by increasing carcass deadfall. Previous studies that evaluate the effectiveness of reducing fishing and establishing marine protected areas to address climate change suggest that these efforts support climate adaptation8.CDR Potential
Estimated Sequestration Potential: Unclear, due to large knowledge gaps around biomass estimates of marine fish stocks, uncertainties in direct and indirect carbon export and overall carbon fluxes, few studies observing natural senescence / dead falls of fishes, and a poor understanding of the life cycle emissions of most fish species9.
Sequestration Durability: Unknown.
Challenges
- More research is needed to understand carbon fluxes and life cycle emissions before developing strategies to increase carbon uptake and sequestration.
Key Knowledge Gaps
Adapted from EDF Natural Climate Solutions in the Open Ocean
- Lack of knowledge around the natural senescence or resulting deadfalls of fishes
- What is the contribution of fishes to inorganic carbon export via carbonate excretion?
- What is the total biomass of fishes, the fluxes in these communities, and conversion factors to go from biomass to units of carbon?
- How to discern between active carbon transport from mesopelagic fish versus from zooplankton?
- What is the composition of mesopelagic communities and what are the differences in roles played by small fishes versus other species such as cephalopods?
First-Order Priorities
*Adapted from EDF Natural Climate Solutions in the Open Ocean
- Conduct studies observing the natural senescence or resulting deadfalls of fishes*
- Conduct additional studies to understand the contribution of fishes to inorganic carbon export via carbonate excretion10*
- Create new models, observing technologies, and data to better constrain the total biomass of fishes, the fluxes in these communities, and conversion factors to go from biomass to units of carbon*
- Create new observational methods and models to better discern between active carbon transport from mesopelagic fish versus from zooplankton*
- Conduct studies to better understand the composition of mesopelagic communities to determine the difference in roles played by small fishes versus other species such as cephalopods*
- Sarmiento, Jorge L., and Nicolas Gruber. 2006. “Organic Matter Production.” In Ocean Biogeochemical Dynamics, 117–72. Princeton, N.J.: Princeton University Press.
- Wilson, Rod W., F. J. Millero, J. R. Taylor, P. J. Walsh, V. Christensen, S. Jennings, and M. Grosell. 2009. “Contribution of Fish to the Marine Inorganic Carbon Cycle.” Science 323 (5912): 359–62. doi.org/10.1126/ science.1157972.
- Boyd, Philip W., Hervé Claustre, Marina Levy, David A. Siegel and Thomas Weber. 2019. “Multi-Faceted Particle Pumps Drive Carbon Sequestration in the Ocean.” Nature 568 (7752): 327–35. doi.org/10.1038/s41586-019-1098-2.
- Mariani, Gaël, William W. L. Cheung, Arnaud Lyet, Enric Sala, Juan Mayorga, Laure Velez, Steven D. Gaines, Tony Dejean, Marc Troussellier, and David Mouillot. 2020. “Let More Big Fish Sink: Fisheries Prevent Blue Carbon SequestrationHalf in Unprofitable Areas.” Science Advances 6 (44): eabb4848. doi.org/10.1126/sciadv.abb4848.
- Bianchi, Daniele, David A. Carozza, Eric D. Galbraith, Jérôme Guiet, and Timothy DeVries. 2021. “Estimating Global Biomass and Biogeochemical Cycling of Marine Fish with and without Fishing.” Science Advances 7 (41): eabd7554. doi.org/10.1126/sciadv.abd7554.
- Saba, Grace K., Adrian B. Burd, John P. Dunne, Santiago Hernández-León, Angela H. Martin, Kenneth A. Rose, Joseph Salisbury, et al., 2021. “Toward a Better Understanding of Fish-Based Contribution to Ocean Carbon Flux.” Limnology and Oceanography 66 (5): 1639–64. doi.org/10.1002/ lno.11709.
- Wilson, Rod W., F. J. Millero, J. R. Taylor, P. J. Walsh, V. Christensen, S. Jennings, and M. Grosell. 2009. “Contribution of Fish to the Marine Inorganic Carbon Cycle.” Science 323 (5912): 359–62. doi.org/10.1126/ science.1157972.
- J.-P. Gattuso, A. K. Magnan, L. Bopp, W. W. L. Cheung, C. M. Duarte, J. Hinkel, E. McLeod, F. Micheli, A. Oschlies, P. Williamson, R. Bille, V. I. Chalastani, R. D. Gates, J. O. Irisson, J. J. Middelburg, H. O. Portner, G. H. Rau, Ocean solutions to address climate change and its effects on marine ecosystems. Front. Mar. Sci. 5, 337 (2018).
- Collins, J. R., Boenish, R. E., Fujita, R. M., Rader, D. N., and Moore, L. A. 2022. Natural climate solutions in the open ocean: scientific knowledge and opportunities surrounding four potential pathways for carbon dioxide removal or avoided emissions. Environmental Defense Fund, New York, NY. https://www.edf.org/sites/default/files/2022-10/Natural%20Climate%20Solutions%20in%20the%20Open%20Ocean.pdf
- Salter, Michael A., et al. “Calcium carbonate production by fish in temperate Marine Environments.” Limnology and Oceanography, vol. 64, no. 6, 2019, pp. 2755–2770, https://doi.org/10.1002/lno.11339.
Environmental Co-Benefits of Animal Carbon
- Nutrient cycling1
- Restoring the role of marine organisms in the carbon cycle2
- Ratnarajah L, Bowie AR, Lannuzel D, Meiners KM, Nicol S (2014) The Biogeochemical Role of Baleen Whales and Krill in Southern Ocean Nutrient Cycling. PLoSONE9(12):e114067. doi:10.1371/journal.pone.0114067
- National Academies of Sciences, Engineering, and Medicine 2022. A Research Strategy for Ocean-based Carbon Dioxide Removal and Sequestration. Washington, DC: The National Academies Press. https://doi.org/10.17226/26278.
Environmental Risks of Animal Carbon
Social Co-Benefits of Animal Carbon
Restoration of marine animal populations may generate many benefits for surrounding communities and economies. While we are calling these “co-benefits” here, it should be noted that some of these benefits may be so significant (while carbon sequestration feasibility and efficacy remain unknown) that these may in fact be the main benefits of restoration. Many of the environmental benefits relate directly to social benefits, such as fisheries enhancement and coastal protection. (Note that there are significantly more co-benefits in “blue carbon” than any of the other CDR Road Maps.)
- Potential for economic stimulation and job creation from enhanced tourism (e.g., whale watching - of particular value to coastal communities that are dependent on marine-based livelihoods)
- Cultural / intrinsic / recreational value
- Education and research – the pursuit of restoring blue carbon for CDR will naturally add value to the existing body of research and can provide valuable educational experiences for the upcoming generation of scientists and conservationists.
Social Risks of Animal Carbon
Efforts around restoration efforts are often met with social acceptance and public support, especially when compared to other climate solutions seen as “tampering with nature”1. This may be in part due to the minimal risks (real and perceived) associated with restoration efforts. While some risks, outlined below, do exist, there may be a higher tolerance for such risks given the long list of potential benefits and co-benefits (both environmentally and socially).
- Potential for negative economic impacts to some industries such as oil and gas, fisheries, and mining2
- Stricter fisheries management to increase species abundances can cause impacts on food security, displacement, and economic strain. (for example, Marine Protected Areas can cause varied impacts among communities and social groups and can be met with opposition)3
- Wolske, K. S., K. T. Raimi, V. Campbell-Arvai, and P. S. Hart. 2019. “Public support for carbon dioxide removal strategies: the role of tampering with nature perceptions.” Climatic Change 152 (3-4):345-361. doi:10.1007/s10584-019-02375-z.
- National Academies of Sciences, Engineering, and Medicine 2022. A Research Strategy for Ocean-based Carbon Dioxide Removal and Sequestration. Washington, DC: The National Academies Press. https://doi.org/10.17226/26278.
- Mascia, M. B., C. A. Claus, and R. Naidoo. 2010. Impacts of marine protected areas on fishing communities. Conservation Biology 24(5):1424-1429. doi:10.1111/j.1523-1739.2010.01523.x.
Common Governance: Policy Opportunities & Challenges
Overview
Some restoration efforts (particularly those most studied such as mangroves and salt marshes) are generally feasible, local, and have high local governability1, however the scale and durability of these efforts will be critical to their success as effective CDR pathways. The restoration of marine habitats and species aligns with several broad-reaching international agreements and domestic laws2. Several international agreements and policies that recognize the value and importance of restoring marine life and ecosystems and that may be important when considering scaling up restoration efforts include:
- Parties to the United Nations Convention on the Law of the Sea (UNCLOS)
- The Convention on Biological Diversity (CBD)
- The United Nations Decade of Ecosystem Restoration (2021-2030)
- UNFCCC Paris Agreement
- Coastal ecosystems can be included in Nationally Determined Contributions (NDCs)
- Global Biodiversity Framework
- Commission for the Conservation of Antarctic Marine Living Resources (CCAMLR)
- UN High Seas Treaty (BBNJ Treaty)
- The Habitats Directive3 (European Commission)
Examples of agreements aimed at protecting marine life
- The Endangered Species Act (United States)
- The Marine Mammal Protection Act (United States)
- The National Marine Sanctuaries Act (United States)
- Marine Protected Areas (Note that MPAs are designed to protect and conserve biodiversity, rather than carbon. For coastal systems, these will be located in national or state waters.)
Examples of US Blue Carbon Policy
- Executive Order 14008: Tackling the Climate Crist at Home and Abroad (2021): Places the climate crisis at the forefront of foreign policy and national security planning.
- America the Beautiful Initiative: Outlines inclusive and collaborative plan for locally led efforts to conserve, steward, and restore land and waters.
- Justice 40 Initiative: A national commitment to environmental justice which includes investments in the reduction of legacy pollution.
- Ocean Climate Action Plan (OCAP): Includes ongoing and planned federal ocean-based climate mitigation and adaptation activities and recommends new or enhances ocean science and policy actions to tackle climate change. It also includes environmental justice considerations into ocean-based climate solutions.
- CREST Act of 2023: An expansion on the current research and development programs of the Department of Energy for capturing and storing carbon dioxide to include methods like CDR.
- Coastal Habitat Conservation Act of 2023: Authorizes the appropriation of $111.5 million over the 2024-2028 period for the US Fish and Wildlife Service to implement the Coastal Program.
- Gattuso, J.-P., A. K. Magnan, L. Bopp, W. W. L. Cheung, C. M. Duarte, J. Hinkel, E. McLeod, F. Micheli, A. Oschlies, P. Williamson, R. Billé, V. I. Chalastani, R. D. Gates, J. O. Irisson, J. J. Middelburg, H. O. Pörtner, and G. H. Rau. 2018. Ocean solutions to address climate change and its effects on marine ecosystems. Frontiers in Marine Science 5:337. doi:10.3389/fmars.2018.00337.
- National Academies of Sciences, Engineering, and Medicine 2022. A Research Strategy for Ocean-based Carbon Dioxide Removal and Sequestration. Washington, DC: The National Academies Press. https://doi.org/10.17226/26278.
- https://environment.ec.europa.eu/topics/nature-and-biodiversity/habitats-directive_en
Opportunities
- Prioritize leadership and partnership with local communities and recognize formal and informal tenure agreements.
- Networks of nations (e.g., International Partnership for Blue Carbon) can aid in building capacity to implement policies that support blue carbon projects.
- New IPCC inventory guidance is needed on emissions inventories for climate mitigation resulting from restoring seagrass, seaweed, and other blue carbon ecosystems (the current lack of guidance here also limits private sector investment)
Challenges
- Property rights, coastal and offshore, are often unclear, not well defined, or not enforced, begging the question, who has jurisdiction over what. This is further complicated by the lateral movement of carbon in blue carbon ecosystems.
- Local governance of global commons is further complicated by local contexts, management structures, and benefit-sharing rules1.
- Permitting may also pose an additional obstacle for some restoration projects.
- Regulations for coastal developments could impede coastal vegetated ecosystem restoration and limit the ability to launch larger restoration projects.
- Merk, Christine; Grunau, Jonas; Riekhof, Marie-Catherine; Rickels, Wilfried (2022) : The need for local governance of global commons: The example of blue carbon ecosystems, Kiel Working Paper, No. 2201, Kiel Institute for the World Economy (IfW), Kiel
Additional Resources
General
- Pacific Northwest Blue Carbon Working Group
- Coastal Carbon Research Coordination Network
- International Partnership for Blue Carbon
- The Blue Carbon Initiative
- The Blue Carbon National Working Group
- Blue Carbon for Our Planet Act
- The Blue Boat Initiative
- Blue Carbon Network (Pew)
- Blue Carbon Lab (Deakin University)
- Blue Carbon Canada (UN Decade Action)
- Global Mangrove Watch
- Blue Carbon Calculator
- Blue Carbon Explorer (TNC) (Map)
- Coastal Carbon Atlas
- Blue Carbon Action Partnership
- Global Ocean Decade Programme for Blue Carbon (UN Decade Program)
- Blue Ventures (GEM etc)
- Blue Carbon Accelerator Fund
- Sea4soCiety
Examples of Mangrove Forest Restoration Efforts
- Delta Blue Carbon (Pakistan)
- Global Mangrove Alliance
- Mangrove Action Project (US)
- Blue Carbon Lab (Australia)
- Vida Manglar Carbon Project (Colombia)
Examples of Seagrass Restoration Efforts
- Ocean Alive (Portugal)
- MedGardens (Mallorca)
- ResilienSEA (West Africa)
- Ozfish (Australia)
- Project Seagrass (UK & Globally)
- For an example of a successful seagrass bed restoration, see Orth et al. 20201.
- https://www.science.org/doi/10.1126/sciadv.abc6434
Examples of Salt Marsh Restoration Efforts
Growing and Maintaining Public Support
Overview
Overview
Successful adoption of any new technology or innovation seldom relies on its function, engineering, or design alone. Some level of public awareness and acceptance is needed for uptake of any new technology; technologies associated with ocean-based carbon dioxide removal (CDR) are not an exception.
Participants in the workshops and review cycles leading to the production of these road maps broadly agreed that ocean-based CDR technology pathways are currently at very low levels of public awareness and, accordingly, public support. These views are in broad agreement with the growing scholarly research on the subject.1
Given these low levels of awareness and understanding, workshop participants agreed that a great deal more investment is needed to educate and inform key audiences on ocean-based CDR, and to connect directly to those key audiences that will be most critical to accelerate the research and development of ocean-based CDR.
- Cox E, Boettcher M, Spence E and Bellamy R (2021) Casting a Wider Net on Ocean NETs. Front. Clim. 3:576294. doi: 10.3389/fclim.2021.576294
Barriers to Increasing Public Acceptance
“Vicious Cycle” of Low Investment/Low Awareness
For some technologies, public awareness and technological readiness can exist in a virtuous cycle, wherein successful development of said technology (e.g., smart phones) increases public attention and support which in turn further accelerates support for more development. The converse is also true. In a “vicious cycle”, low levels of development lead to low levels of awareness which in turn inhibits flow of resources even for research and development.
Many ocean-based pathways are trapped in this latter cycle, and hence remain critically underdeveloped.
A few points of evidence include:
- The relative paucity of information about ocean-based CDR pathways relative to terrestrial and technological pathways in CarbonPlan’s CDR database.1
- The paucity of ocean-based applications submitted to Stripe’s first round of negatives emissions purchases2 and Microsoft’s first negative emissions purchase.3
- The lack of big environmental and climate NGOs that include ocean-based CDR, or any CDR for that matter, as priorities on their climate or ocean agendas.
- The minimal attention paid to ocean-based CDR pathways in the IPCC’s Special Report on the Ocean and Cryosphere in a Changing Climate4 suggests a lack of scientific awareness that further slows broader public awareness.
- “Carbonplan / Reports.” Carbonplan, carbonplan.org/reports. (as of Feb. 2021)
- Stripe. “Stripe/Negative-Emissions-Source-Materials.” GitHub, github.com/stripe/negative-emissions-source-materials; ocean-based submissions for the second round of purchases did increase (https://github.com/stripe/carbon-removal-source-materials/tree/master/Project%20Applications/Spring2021)
- Microsoft carbon removal: Lessons from an early corporate purchase. 2021. https://query.prod.cms.rt.microsoft.com/cms/api/am/binary/RE4MDlc
- Bindoff, N.L., W.W.L. Cheung, J.G. Kairo, J. Arístegui, V.A. Guinder, R. Hallberg, N. Hilmi, N. Jiao, M.S. Karim, L. Levin, S. O’Donoghue, S.R. Purca Cuicapusa, B. Rinkevich, T. Suga, A. Tagliabue, and P. Williamson, 2019: Changing Ocean, Marine Ecosystems, and Dependent Communities. In: IPCC Special Report on the Ocean and Cryosphere in a Changing Climate [H.-O. Pörtner, D.C. Roberts, V. Masson-Delmotte, P. Zhai, M. Tignor, E. Poloczanska, K. Mintenbeck, A. Alegría, M. Nicolai, A. Okem, J. Petzold, B. Rama, N.M. Weyer (eds.)]. In press.
The “Moral Hazard” of CDR Argument
Another cause of the low levels of development of ocean-based CDR relates to what is referred to as the “moral hazard” of CDR itself.
CDR, whether in the oceans or on land, has been dubbed by some as a “moral hazard” that will detract energy and attention away from the [more] important effort to reduce current and future greenhouse gas emissions across all sectors of society1. The fear is that some actors will use CDR as a means to reduce their net emissions, while avoiding more difficult reductions in gross greenhouse gas emissions.
Even though the international science community now recognizes that we need both CDR and overall emission reductions to stabilize planetary warming at 1.5ºC above pre-industrial levels23, understanding and stakeholder recognition of the imperative for CDR remains low, and the “moral hazard” argument is partly responsible.
- Lawford-Smith H, Currie A. 2017 Accelerating the carbon cycle: the ethics of enhanced weathering. Biol. Lett. 13: 20160859. http://dx.doi.org/10.1098/rsbl.2016.0859
- IPCC, 2018: Summary for Policymakers. In: Global Warming of 1.5°C. An IPCC Special Report on the impacts of global warming of 1.5°C above pre-industrial levels and related global greenhouse gas emission pathways, in the context of strengthening the global response to the threat of climate change, sustainable development, and efforts to eradicate poverty [Masson-Delmotte, V., P. Zhai, H.-O. Pörtner, D. Roberts, J. Skea, P.R. Shukla, A. Pirani, W. Moufouma-Okia, C. Péan, R. Pidcock, S. Connors, J.B.R. Matthews, Y. Chen, X. Zhou, M.I. Gomis, E. Lonnoy, T. Maycock, M. Tignor, and T. Waterfield (eds.)]. In Press.
- IPCC, 2022: Summary for Policymakers. In: Climate Change 2022: Mitigation of Climate Change. Contribution of Working Group III to the Sixth Assessment Report of the Intergovernmental Panel on Climate Change [P.R. Shukla, J. Skea, R. Slade, A. Al Khourdajie, R. van Diemen, D. McCollum, M. Pathak, S. Some, P. Vyas, R. Fradera, M. Belkacemi, A. Hasija, G. Lisboa, S. Luz, J. Malley, (eds.)]. Cambridge University Press, Cambridge, UK and New York, NY, USA. doi: 10.1017/9781009157926.001
Low Awareness of the Ocean’s Potential for Carbon Removal
Even when CDR is viewed as a critical tool, the role of the ocean lags far behind other pathways in public understanding and acceptance. This despite the fact that the ocean covers ~71% of the surface area of the planet, and already plays a major planetary role in cycling atmospheric and terrestrial carbon and safely storing it in the deep sea.
Despite the ocean’s potential for efficacious and scalable carbon removal, ocean-based pathways have not been investigated with the same level of interest and rigor as land-based (e.g., afforestation), technological (e.g., direct air capture), and hybrid (e.g., bioenergy with carbon capture and storage) approaches. For example, an earlier report on CDR1 pathways by the Academy largely ignored ocean-based pathways (with the exception of restoration of coastal aquatic vegetative habitats)2. We hope that the call for about $1.3 billion dollars over ten years in new prioritized research and development in the recently released (2022) U.S. National Academy of Sciences publication of the research strategy for ocean-based CDR will help to increase the awareness of the ocean's potential for carbon removal.
- National Academies of Sciences, Engineering, and Medicine. (in progress). A Research Strategy for Ocean Carbon Dioxide Removal and Sequestration. https://www.nationalacademies.org/our-work/a-research-strategy-for-ocean-carbon-dioxide-removal-and-sequestration
- National Academies of Sciences, Engineering, and Medicine. 2019. Negative Emissions Technologies and Reliable Sequestration: A Research Agenda. Washington, DC: The National Academies Press. doi: https://doi.org/10.17226/25259.
Public Perceptions About Relative Environmental Risks
The ongoing and future threats to marine ecosystems from both legacy and continuing greenhouse gas pollution are clear and urgent. They include sea level rise and unprecedented rates of warming and acidification that threaten the existence of critical ocean ecosystems, such as coral reefs and kelp forests, as well as severe alteration to marine biodiversity and ecosystem function generally1.
The harms posed by a warming, rising, and acidifying ocean are already clear and visible, especially for island and coastal countries. Polling among coastal residents of the United States done in 2022 also shows concern about the impact of climate change in the ocean and support for ocean-based carbon dioxide removal. However, for many people the terrestrial impacts - increased wildfires, stronger hurricanes, and heatwaves and drought on land- are more visible and may make the climate crisis appear more dire on land than in the ocean.
In spite of the clear impacts of and continuing risks posed by the build-up of carbon dioxide pollution in the air and water, there seems to be more fear about the risks of taking experimental action versus the risks of inaction. This is particularly true for ocean pathways, with a reticence as some have stated to “use the ocean to fix the climate” (which overlooks the fact that they are inextricably intertwined).
There are concerns about unknown and unbounded environmental risks from ocean-based CDR pathways, many of which may have been born from controversies over ocean iron fertilization experiments in the 1990s and 2000s23 or the ocean fertilization project by the Haida Salmon Restoration Corporation off Canada4.
- IPCC, 2019: Summary for Policymakers. In: IPCC Special Report on the Ocean and Cryosphere in a Changing Climate [H.-O. Pörtner, D.C. Roberts, V. Masson-Delmotte, P. Zhai, M. Tignor, E. Poloczanska, K. Mintenbeck, A. Alegría, M. Nicolai, A. Okem, J. Petzold, B. Rama, N.M. Weyer (eds.)]. In press.
- Strong, A.L., J.J. Cullen, and S.W. Chisholm. 2009. Ocean fertilization: Science, policy, and commerce. Oceanography 22(3):236–261, https://doi.org/10.5670/oceanog.2009.83.
- Strong, A., Chisholm, S., Miller, C. et al. Ocean fertilization: time to move on. Nature 461, 347–348 (2009). https://doi.org/10.1038/461347a
- Tollefson, J. ‘Plankton-boosting project in Chile sparks controversy’, p. 2. Nature 545, 393–394 (25 May 2017) doi:10.1038/545393a
The Precautionary Principle/”No Action Fallacy”
The precautionary principle has often been used to manage risk when a proposed activity has the potential for causing harm, and where extensive scientific knowledge and conclusive evidence to the contrary is lacking. Implicit in the precautionary principle is that the burden of proof falls on the proponent of the action to show that potential harm can be avoided or minimized.
Also implicit is that potential harm can be avoided by not taking the action. However, this assumes that the condition of the system will stay constant without the action. This is certainly not the case in the oceans; there is overwhelming scientific evidence that the ocean is on a dangerous downward trajectory as a result of too much carbon in the air and water1.
If one accepts the foundational premise that “no action” on CDR is not a credible alternative, then any ocean-based CDR action has to be compared against other CDR actions that will equally solve for the problems in the ocean driven by high levels of greenhouse gases in the air and water. It is not credible to compare ocean-based CDR to a “no-action” alternative: inaction is not “safe”, and a “no-action alternative” is a fallacy as a choice to safeguard marine biodiversity and ecosystems. No action leads to continuing loss of ocean health and growing threats to communities and economies.
- Malhi Y, Franklin J, Seddon N, Solan M, Turner MG, Field CB, Knowlton N. 2020 Climate change and ecosystems: threats, opportunities and solutions. Phil. Trans. R. Soc. B 375: 20190104. http://dx.doi.org/10.1098/rstb.2019.0104
Perceptions on Nature-Based versus Engineered Solutions
Although ocean-based CDR technologies are nascent, there is already emerging evidence of public preference for pathways regarded as more “nature-based”, such as restoration of coastal blue carbon habitats, over “engineering-based” approaches, such as ocean alkalinity enhancement. This appears to be due to concerns about the potential for environmental risk and unintended impacts with “engineering-based” approaches.
This apparent bias for nature-based approaches creates a situation where the techniques with greatest CDR potential and permanence (“engineering-based”) face greater obstacles to public acceptance than those with reduced CDR potential and permanence (“nature-based”)1.
- Bertram C and Merk C (2020) Public Perceptions of Ocean-Based Carbon Dioxide Removal: The Nature-Engineering Divide? Front. Clim. 2:594194. doi: 10.3389/fclim.2020.594194
Underdeveloped Regulatory and Governance Structures
Advancing the development and testing of ocean-based CDR approaches will require governance structures that both enable the permitting of legitimate testing and development and ensure that the public interests are protected.
Current governance-related challenges include:
- There are no specific regimes in the US nor internationally governing ocean-based CDR1.
- In many countries, there are complex regulatory mazes to navigate to gain permits for in-water experimental trials, hindering development of trials.
- In the US this is particularly complicated by overlapping jurisdictions and authorities in the coastal zone2.
These and other governance and regulatory uncertainties present real challenges and risks to those working to conduct research and development on ocean-based CDR pathways. Lack of such regimes both inhibits experimentation and the development of public confidence in ocean-based CDR experimentation. Governance structures and regimes with a specific focus on ocean-based CDR must be developed.
These governance structures must provide consistency; transparency; work to facilitate experimentation and demonstration; minimize negative environmental impacts; and ensure that field trials are controllable in size and scope. They may also require different skill sets appropriate to specific ocean CDR pathways (i.e. different governance for macroalgal sequestration versus ocean liming)3.
- REMOVING CARBON DIOXIDE THROUGH OCEAN ALKALINITY ENHANCEMENT AND SEAWEED CULTIVATION: Legal Challenges and Opportunities By Romany M. Webb, Korey Silverman-Roati, and Michael B. Gerrard February 2021 - Working Draft.
- Suatoni, Lisa. (2021) ‘Remarks at The National Academies of Sciences, Engineering, and Medicine: A Research Strategy for Ocean Carbon Dioxide Removal and Sequestration: Workshop Series, Part 2 ’. 27-January-2021.
- Bellamy, R. Incentivize negative emissions responsibly. Nat Energy 3, 532–534 (2018). https://doi.org/10.1038/s41560-018-0156-6
First Order Priorities
Broaden and Strengthen Base of Support for Ocean-Based CDR
It is essential to swiftly raise awareness of and knowledge about ocean-based CDR across the key sectors and actors that are engaged in shaping climate and ocean policy. As various alternatives are evaluated, ocean-based CDR needs a “seat at the table” and to receive due consideration. This requires a much broader array of voices articulating the case for consideration than we currently have. It is a priority to engage a broad and diverse range of credible individuals, organizations and entities to vocally support research and development of ocean-based CDR.
The key first step is to design and launch a multi-dimensional, multi-year communications campaign to reach critical actors in this space.
- Target actors include policymakers at multiple levels, climate and ocean specialists, marine sectors, scientists, investors, philanthropists, entrepreneurs and others. The campaign should be designed to educate about the outsize and growing impacts of greenhouse gas pollution on the oceans, the role of CDR as one approach to slowing these impacts, and the potential role of ocean-based pathways for CDR. All with the underlying objective to expand support for accelerated research, development and demonstration of ocean-based CDR pathways.
The communications campaign should:
-
-
- Identify all target audiences that will be part of creating an enabling environment for ocean-based CDR RD&D
- Conduct research on their current perceptions and understanding of ocean-based CDR.
- Develop appropriate content and information formats for these key audiences.
- Identify and engage credible spokespeople (scientists, business leaders, other opinion leaders) to help carry the messages1.
-
- As an example of the power of well-positioned spokespeople, look to the recently announced Carbon Removal XPrize funded by Elon Musk https://www.xprize.org/prizes/elonmusk
Ensure Broad Public Engagement in All Field Trials
Ocean-based CDR field trials will stand the best chance of success when they are developed with as many communities of interest participating as possible. All practitioners and researchers need to move field trials forward with transparency and broad stakeholder and interest group involvement, and with third-party scientific review and validation. It is particularly important to expand access for diverse communities of interest to participate in the development of this field.
Researchers and practitioners need to:
- Cultivate alliances with diverse stakeholders:
- Communities subjected to ocean change, including fishers and tribal communities.
- Indigenous and tribal communities who often hold legal rights to the sea and marine resources.
- Work with partners already trusted by communities (e.g., SeaGrant in the US).
- Work with local stakeholders to ensure that benefits of field testing (environmental and economic) are equitably shared, and that risks of field testing are not externalized on local communities.
- Ensure there are adequate resources as part of field trial designs to engage stakeholders.
- Identify and quantity co-benefits to the environment and people of ocean-based CDR approaches.
- Engage social scientists to design involvement processes and help address economic, social, and political challenges and opportunities around ocean-based CDR.
- Ensure broad dissemination of progress and results.
Develop a code of conduct to guide scientific experimentation and field trials.
- Engage relevant stakeholders in development of a code1 to guide scientific experimentation and field trials in a manner that is transparent, participatory, rigorously monitored and carefully controlled.
- The Aspen Institute, with support from the ClimateWorks Foundation, published a set of key questions that should be considered by researches and practitions of ocean-based CDR.
- Develop assessment frameworks to guide scientific field trials under all relevant international conventions and national laws.
- Hubert, A.‐M. (2021), A Code of Conduct for Responsible Geoengineering Research. Glob Policy. https://doi.org/10.1111/1758-5899.12845
Engage Governments
-
Government actors at various levels responsible for climate action need to be specifically engaged to ensure that ocean-based CDR is considered in national programs for climate mitigation. There requires increasing governmental awareness and support for ocean-based CDR RD&D at national and international scales.
- Increase outreach to relevant political jurisdictions to educate about ocean-based CDR opportunities and needs.
- Seek to expand governmental involvement in ocean-based CDR through enabling legislation and policy.
- Work with relevant jurisdictions to expand governmental support/funding for needed RD&D.
- Build a broad coalition/alliance to demonstrate the diversity of interests in support of ocean-based CDR testing.
Develop Effective Regulatory & Governance Structures
-
Sub-national, national, and international regimes to govern development and testing of ocean-based CDR are absolutely critical for this field to progress. Key first order needs are:
- Governance Reviews: Governance reviews are needed to identify and describe the existing legal frameworks for ocean-based CDR pathways in priority coastal countries/jurisdictions.
- The Sabin Law Center at Columbia University has initiated such an effort with working papers to look at legal challenges and opportunities for:
- Macroalgal cultivation and sequestration and ocean alkalinity enhancement in the US1.
- Offshore carbon capture and storage in Canada2
- The legal framework for sub-seabed carbon storage in the U.S and Canada 34
- The Sabin Law Center at Columbia University has initiated such an effort with working papers to look at legal challenges and opportunities for:
Planned additional working papers will add geographic context and expand the suite of ocean-based CDR pathways considered.
- Identify Needed Improvements to Governance Regimes
- Review scans to assess gaps, needs, and opportunities.
- Convene stakeholders at relevant scales to discuss and develop draft proposals for new or amended regimes, including developing specific legislative and executive directives that provide key agencies with scope to engage in ocean-based CDR.5
- Engage with sub-national jurisdictions. Small-scale field testing under the jurisdiction of cities and/or states may help build data and evidence to support larger-scale testing in federal waters and under federal authority.
- Review and Propose Needed Changes to International Regimes
- Engage a global community of experts to review existing international governance regimes and laws for ocean-based CDR RD&D, and to make specific recommendations for improving the international regimes.
- Identify the specific processes and opportunities to more clearly embed governance and oversight of ocean-based CDR into international governance frameworks.
- Engage a global community of experts to review existing international governance regimes and laws for ocean-based CDR RD&D, and to make specific recommendations for improving the international regimes.
-
- Develop Tools to Help Researchers and Experimental Efforts
- Permitting guides: Develop specific tools and plans by country (and sub-national jurisdictions) to navigate the leasing, permitting, and legal considerations.
- Convene relevant practitioners in working groups to identify and advocate for common tools and information needs that would benefit all practitioners of a given methodology (e.g. coastal enhanced weathering) in a specific jurisdiction.
- Co-develop such tools with the relevant permitting agencies to “bring regulators along for the journey”.
- Identify and support development of the needed interagency and intergovernmental structures to facilitate review and compliance.
- Governance Reviews: Governance reviews are needed to identify and describe the existing legal frameworks for ocean-based CDR pathways in priority coastal countries/jurisdictions.
- Webb, R.; Silverman-Roati, K. and Gerrard, M. (2021). Removing Carbon Dioxide Through Ocean Alkalinity Enhancement and Seaweed Cultivation: Legal Challenges and Opportunities. Sabin Center for Climate Change Law, Columbia Law School. https://ssrn.com/abstract=3800494
- Webb, R. and Gerrard, M. (2021). The Legal Framework for Offshore Carbon Capture and Storage in Canada. Sabin Center for Climate Change Law, Columbia Law School. https://ssrn.com/abstract=3800493
- Webb, R and Gerrard, M. (2017). Policy Readiness for Offshore Carbon Dioxide Storage in the Northeast. Sabin Center for Climate Change Law, Columbia Law School. https://ssrn.com/abstract=2986463
- Webb, R and Gerrard, M. (2019) Overcoming Impediments to Offshore Carbon Dioxide Storage: Legal Issues in the U.S. and Canada. Sabin Center for Climate Change Law, Columbia Law School. https://ssrn.com/abstract=3359754
- Energy Futures Initiative (2020). Uncharted Waters: Expanding the Options for Carbon Dioxide Removal in Coastal and Ocean Environments. https://static1.squarespace.com/static/58ec123cb3db2bd94e057628/t/6011a63f65321405af54681f/1611769420810/Uncharted+Waters.pdf.pdf
Increase Practitioners in Ocean-based CDR RD&D
-
It is essential to expand the field of researchers from across diverse disciplines to engage on ocean-based CDR-related research. Many of the above activities to legitimize the field will help draw more scholarly attention but additional actions could also help, such as:
- Create prize competitions, awards, and funding opportunities to mobilize ocean scientists and engineers to work on critical needs identified throughout these road maps.
- Develop long-term strategies to engage more leading academic and research institutions against these challenges and opportunities to build the next generation of researchers and practitioners.
Expanding Finance and Investment
Overview
Overview
One of the most critical gaps in further exploration, development, and testing of ocean-based CDR technologies is the very limited amount of financial resources being invested into the space.
Without exception, all ocean-based CDR technologies examined in these road maps need substantial increases in public, philanthropic, and private investment to accelerate technology development, de-risk technologies, and develop monitoring, reporting, and verification protocols. The global community of experts who participated in the scoping workshops for these road maps broadly agreed that ocean-based CDR pathways remain substantially underinvested given their potential to contribute to gigaton-scale, affordable CDR.
Critical Obstacles and Development Needs
“Vicious Cycle” of Low Investment/Low Awareness
The lack of awareness of and support for ocean-based CDR amongst key audiences was clearly identified as one of the (if not the) primary obstacles to unlocking greater resources that could accelerate the development and testing of ocean-based CDR pathways. Readers may consult the road map “Growing & Maintaining Public Support” which details the specific obstacles, needs, and opportunities to advance public awareness and support.
Participants in the expert workshops leading to the production of this road map broadly agreed that there exists a “vicious cycle” whereby the lack of investments into ocean-based CDR pathways has prevented proof-of-concept field trials from taking place. These field trials are in turn critical to answer key questions on environmental benefits and risks, as well as technical feasibility, which in turn could increase enhance public confidence in further exploration of ocean-based CDR pathways.
Without this essential information, and/or without increasing public support, there is likely to be continued underinvestment into pilot projects.
Minimal Levels of Public Investment to Date
Public investment from governments must play a critical role in providing early-stage funding to development and field testing of ocean CDR technologies, which in turn helps to “de-risk” private investment and finance of ocean-based CDR efforts. This public investment de-risking function is critical to create the enabling conditions for building an industry and a market and has been done successfully in a number of fields, from healthcare to renewable energy. So far, public investment in ocean- based CDR is lacking.
A previous analysis by the Energy Futures Initiative and the Bipartisan Policy Center of US federal investments in ocean-based CDR RD&D found a total expenditure of only $44.3 million on marine pathways across 115 projects from 2005 to 20181.
In the US, the US Department of Energy’s Advanced Research Projects Agency – Energy (ARPA-E) is the primary source of US public support through the $50 million MARINER program designed “to develop the tools to enable the United States to become a global leader in the production of marine biomass” (but for energy, not CDR) and through $2 million in recently announced awards to support research and development on direct ocean capture (electrochemical) technologies.
In the EU, the OceanNETs (NETs: Negative Emission Technologies) project is evaluating a wide range of ocean-based CDR pathways for their theoretical, technological, economic, social, and political viability. OceanNETs is funded at € 7.19 million (~$8.75 million).
While these government funding programs are steps in the right direction, they fall well short of the recently released call for a 10-year, $1.75 billion US federal investment in ocean-based CDR research, development, and demonstration2.
Philanthropic investment is also important to build an enabling environment for increasing public awareness and support of ocean-based CDR pathways3. The ClimateWorks Foundation4 and the Grantham Environmental Trust5 are two of a small number of philanthropic organizations which include ocean-based CDR in their portfolios.
- Hezir, Joseph S., Bushman, Tim, Stark, Dr. Addison K., Smith, Erin. “Carbon Removal: Comparing Historical Federal Research Investments with the National Academies’ Recommended Future” Funding Levels” April 2019.
- Energy Futures Initiative. “Uncharted Waters: Expanding the Options for Carbon Dioxide Removal in Coastal and Ocean Environments.” December 2020.
- Gagern, Antonius. “Demystifying Ocean-Based Carbon Dioxide Removal: An Explainer - Insights from Our Shared Seas.” Our Shared Seas, 19 Jan. 2021. https://oursharedseas.com/demystifying-ocean-based-carbon-dioxide-removal-an-explainer/
- “Climate Works: Ocean Carbon Dioxide Removal (CDR)”. https://www.climateworks.org/programs/carbon-dioxide-removal/oceans/
- Lorenzo, Emanuele Di. “New Partnership to Deploy Ocean-Based Solutions to Climate.” Ocean Visions, 21 Oct. 2020, www.oceanvisions.org/post/new-partnership-with-grantham-environmental-thrust-to-deploy-ocean-based-solutions-to-climate.
Underdeveloped Markets for Carbon Removal
The lack of well-developed markets for carbon removal and permanent storage also affects the flow of early-stage investment into ocean-based CDR pathways specifically.
There has recently been some progress to develop carbon removal markets (e.g. Nori, Puro Earth), and there are a growing number of corporate commitments to carbon removal, alongside several high-profile CDR ‘futures’ purchases from Stripe, Shopify, and Microsoft.
However, more work is needed to keep growing the markets for CDR and to better connect the growing demand for carbon removal to the potential suppliers of carbon removal. More clarity and certainty on the demand side (scale, specifications, pricing, etc.) will give clarity to potential early-stage investors and may increase capital flow into development of the technologies and enterprises that can ultimately deliver carbon removal at a competitive price.
As outlined in the technology-specific road maps (Electrochemical CDR, Macroalgae Cultivation & Carbon Sequestration, Ocean Alkalinity Enhancement), many of the ocean-based CDR pathways also generate co-products that can serve as important sources of revenue for carbon removal ventures. These include, but are not limited to: high value bioproducts, bioplastics, and bioenergy from macroalgae; hydrogen, chlorine, and oxygen gases, as well as hydrochloric acid from electrochemical processes; and valuable metals refined from ocean alkalinity enhancement pathways. Unknowns about the current and projected sizes of the markets for these co-products hinders development of more accurate business models for investors into ocean-based CDR pathways.
Limited Expertise and Labor Force
There was strong agreement in the expert workshops that a shortage of capacity, skills, and knowledge in the ocean CDR field amongst researchers, entrepreneurs, marine managers, and key stakeholders creates obstacles to the enhanced development and testing of ocean-based CDR pathways. More effort is needed to further identify the requisite knowledge and skills set for ocean-based CDR development and to work with relevant institutions to train and develop people with the capacity needed globally,
Technology Development for Needed Tools Still in Early Stages
Investment is needed not only in development of ocean-based CDR technologies but also in the associated tools needed to support the field. This includes a new suite of hardware and software products that can:
- Provide physical, chemical, and biological information at sufficient spatial and temporal density necessary to make informed carbon sequestration estimates.
- Provide remote verification of carbon sequestration and permanence.
- Provide the autonomous or remote monitoring, maintenance, and troubleshooting necessary to support large-scale offshore operations.
Nascent and Uncertain Governance and Regulatory Regimes
Advancing the investment into development and testing of ocean-based CDR approaches will require governance structures that both enable the permitting of legitimate testing and development and ensure that the public interests are protected.
Current governance-related gaps include:
- There are no specific regimes in the US nor internationally governing ocean-based CDR.
- Uncertainty and lack of expertise/knowledge around the jurisdiction and application of relevant international governance regimes to ocean-based CDR (e.g. London Convention/London Protocol, UN Convention on Biological Diversity).
- In many countries, there are complex regulatory mazes to navigate to gain permits for in-water experimental trials, hindering development of trials. This is particularly challenging in countries (e.g. the US) featuring overlapping jurisdictions and authorities in the coastal zone.
These and other governance and regulatory uncertainties present real challenges and risks to those working to conduct research and development on ocean-based CDR pathways and inhibit private investment.
Regimes and protocols around monitoring, reporting, and verification for ocean-based CDR also needs to be strengthened to clarify roles and responsibilities of CDR providers (sellers), buyers, and regulators.
First-Order Priorities
Develop Supportive Governance and Regulatory Regimes for RD&D
Investment in ocean-based CDR will not happen at the scale needed without addressing the current situation of policy and regulatory uncertainty. It is critical to build enabling governance frameworks (a mixture of policy, regulatory structures, and public investment) that provide increasing clarity around how ocean-based CDR can move forward. This must include things like clarified permitting processes, and clearly identified oversight authorities. Detail on key elements of this priority can be found in the Public Support road map.
Develop Needed Foundations for an Ocean-based Carbon Market
A growing carbon market will be ultimately necessary for successful scaling of ocean-based CDR. Ensuring that ocean-based CDR pathways will be accepted into carbon markets will depend upon resolving some critical information challenges in the first instance, including:
- Scope and develop the measurement technologies, and verification tools to support creation and application of standards and protocols for each ocean CDR approach to provide assurances to negative emissions markets. (link to “Develop CDR Monitoring and Verification Protocols” priority in each of the technical maps)
- Develop the science needed to underpin standards for verifying carbon sequestration and permanence from various ocean-based CDR pathways. For example, Oceans2050 is already developing a methodology for quantifying natural carbon sequestration underlying macroalgae farms1, but additional science and research on carbon fate and transport is needed for macroalgae cultivation and sinking.
- “Seaweed Project.” Oceans 2050, www.oceans2050.com/seaweed.
Develop Needed Foundations for an Ocean-based Carbon Market
A growing carbon market will be ultimately necessary for successful scaling of ocean-based CDR. Ensuring that ocean-based CDR pathways will be accepted into carbon markets will depend upon resolving some critical information challenges in the first instance, including:
- Scope and develop the measurement technologies, and verification tools to support creation and application of standards and protocols for each ocean CDR approach to provide assurances to negative emissions markets. (1,2,3)
- Develop the science needed to underpin standards for verifying carbon sequestration and permanence from various ocean-based CDR pathways. For example, Oceans2050 is already developing a methodology for quantifying natural carbon sequestration underlying macroalgae farms1, but additional science and research on carbon fate and transport is needed for macroalgae cultivation and sinking.
- “Seaweed Project.” Oceans 2050, www.oceans2050.com/seaweed.
Connect Corporate Demand for CDR to Ocean-based Pathways RD&D
Corporate buyers of carbon removal (e.g., Stripe, Microsoft) can play a key role in providing support for RD&D needed to make future carbon credit purchases possible.
- Scope creation of a “Carbon Removal Buyers Alliance” to pool demand and resources to help develop ocean-based pathways for carbon removal. Such an Alliance could:
- Provide early developmental and testing funding in exchange for future CDR options
- Provide forward contracts for future purchase of CDR allowing entrepreneurs to make investments now
- Develop and coordinate the specifications needed for the private removal market (as the Renewable Energy Buyers Alliance did for wind and solar). This also would build on existing investments and/or purchase of CDR123, that have allowed for infusions of capital into entrepreneurial efforts needed to further test and develop these approaches.
- Fighting for the Future: Shopify Invests $5M in Breakthrough Sustainability Technologies (no date). Available at: https://news.shopify.com/fighting-for-the-future-shopify-invests-5m-in-breakthrough-sustainability-technologies (Accessed: 7 March 2021).
- Stripe’s first carbon removal purchases (no date). Available at: https://stripe.com/blog/first-negative-emissions-purchases (Accessed: 7 March 2021).
- Stripe’s second carbon removal purchases (May 26, 2021). Available at: https://stripe.com/newsroom/news/spring-21-carbon-removal-purchases (Accessed: 30 June 2021)
Research and Development of Markets for Key Co-Products
Co-products generated from ocean-based CDR pathways need viable routes to market in order to provide revenues that can offset costs of ocean-based CDR deployment. Market analyses need to be performed that evaluate the potential for:
- Existing markets to accommodate additional supply of co-products (e.g. hydrogen gas produced from electrolysis) (Understand Market Potential for Co-Products)
- New markets to grow to match the supply of new co-products with buyers
Accelerate Technology Development
Additional human and physical resources are needed to accelerate ocean-based CDR technology RD&D. Opportunities to accelerate RD&D include:
- Building new public-private partnerships, such as between universities, national laboratories, and industry to address critical bottlenecks in the technological readiness of various ocean-based CDR pathways.
- Cataloging outstanding science questions/gaps pertinent to ocean-based CDR development and working with existing funders of basic and applied science to build funding programs around for the needed science. One such option is encouraging relevant national science funding agencies (e.g., the US National Science Foundation), to open requests for proposals around key science needs.
Enhance Policymaker Awareness and Engagement
Much higher levels of public investment are needed to move ocean-based CDR RD&D forward. This in turn requires a supportive policy environment. Supporters of ocean-based CDR must cultivate awareness and support among policy makers by:
- Developing targeted education and communication strategies for key policymakers at different levels of government to increase support for ocean-based CDR RD&D
- Engaging a diverse coalition of organizations to help educate and inform policy and appropriations for ocean-based CDR RD&D.
Build a Supporting Ecosystem for Ocean-based CDR Entrepreneurs
There are a number of emerging innovators and entrepreneurs working on ocean-based CDR, but they often lack access to the critical talent and expertise needed to move their innovations out of labs and into field trials and potential deployment. There are key actions that can help create a more supportive environment for these entrepreneurs.
- Facilitate related technology transfer out of academia: These road maps can be used to work with specific universities to profile needed technology development and then to help connect academics with external actors. To facilitate better tech transfer, a series of outreach materials and webinars to familiarize ocean scientists and engineers with technology transfer offices at key universities would be useful.
- Build partnerships with leading accelerators, such as Y-Combinator1, the Ocean Solutions Accelerator, Creative Destruction Lab, Larta Institute, or Indie Bio to increase their focus on ocean-based CDR ventures and to access their expertise for building these ventures.
- Work with prizes and competitions, such as those hosted by The Ocean Exchange to ensure inclusion of ocean-based CDR in prizes and competitions.
- Ensure that ocean-based CDR research and development is well represented in the myriad “ocean clusters” springing up (e.g., Alaska Ocean Cluster) to support a global Blue Economy
- “Carbon Removal Technologies.” YC Request for Startups, carbon.ycombinator.com/
Develop Better Tools for Potential Investors
- Develop a publicly available database where people can track investments and projects. Ensure that this includes tracking of public investment in ocean-based CDR, and from countries besides the US and the European Union, to gain a full picture of the global landscape around ocean-based CDR.
- Develop targeted outreach and materials for investor education to build their familiarity and comfort with CDR, and ocean-based CDR specifically (something akin to the Climateworks – Economist knowledge hub for ocean-based CDR pathways). Reach out to specific associations such as TONIIC.
- Develop comparative analyses (e.g., cost-benefit) between ocean-based CDR and terrestrial-based or technology-based CDR to inform and reduce barriers to entry for CDR investors looking to support ocean-based pathways.